Climate change effects and impacts on benthic habitats
Direct and indirect pressures driven by climatic change and ocean acidification factors can significantly alter the environmental conditions (e.g., increase in sea surface temperature, decreases of pH) necessary for benthic ecosystem processes and functions, and therefore affect habitat suitability for sensitive benthic species, species distributions, community structures and diversity patterns (Harley et al., 2006; Hoegh-Guldberg and Bruno 2010; Poloczanska et al., 2013; Gattuso et al., 2015; Nagelkerken and Connell 2015; Poloczanska et al., 2016; Weinert et al., 2016). Climate change could lead to increases in mean sea level rise and changes in storminess, leading to a greater need for flood and coastal erosion defences. There are some uncertainties in the predictions, which will require additional research in order to increase accuracy on the future impacts of benthic habitats, particularly along the coastline.
There is not always a wide range of datasets available to systematically measure the effects of climate change and ocean acidification on benthic habitats and species, due to the paucity of the monitoring programme. However, there is a large volume of evidence of the impacts across different Regions. There are variations in the level and type of impacts due to geography (e.g., Arctic Waters) and the physical characteristics of the seafloor (e.g., depth), and in the typology of benthic habitat composition (e.g., calcifying species).
Benthic habitats also provide solutions for the mitigation and adaption of climate change effects. For example, the natural carbon storage and sequestration capacity of some benthic habitats highlights their major role in the context of climate change and in mitigating the carbon inputs to the atmosphere from human activities. (https://www.equinor.com/energy/northern-lights; https://www.upstreamonline.com/energy-transition/uk-launches-first-ever-carbon-storage-licensing-round/2-1-1237194).
Specific effects of climate change in Region I – Arctic Waters:
Changes in sea-bottom temperature in the western and central Barents Sea have been well documented (e.g., ICES WGIBAR 2021, Skagseth et al., 2020; Weslawski et al., 2010a) (Figure CC.1).
A biogeographic boundary exists in the Barents Sea region, associated with the Polar Front where Atlantic and Arctic water masses meet. Indications of Atlantification (i.e., increased influence by Atlantic water masses) in the region suggest this transition zone will move northward with climate change, which may see Arctic-associated communities retreating north in response (Jørgensen et al., 2014, Buhl-Mortensen et al., 2020).
The most recent ecosystem state report for Arctic Waters (ICES 2021, annexes 4 and 6) describes large-scale stability in distribution, biomass, and abundance patterns in the Region. However, in 2020 the distribution and biomass / abundance of megabenthos were below the long-term mean, with fluctuations being positively correlated to increasing sea temperature.
Temperature preference and bottom depth were included in the baseline analyses of Jørgensen et al., (2022a). While frequencies of cold-water and warm-water taxa were quite similar in Icelandic waters and in North-East Greenland, warm-water taxa were more common in the Barents Sea. Taxa groups found in areas with the coldest median temperature and a relatively narrow temperature range were termed ‘cold-water taxa’ (Figure CC.2). Taxa groups in regions with the warmest median temperature, along with the broadest temperature range, were termed ‘warm-water taxa’.
Jørgensen et al., (2022b) show that in a biogeographic transition zone on the slope north-east of Svalbard, surface deposit feeding crustacean assemblages encounter mobile Arctic echinoderm predators, and that, at a sharp transition some 500 m along the shelf of Svalbard, boreal filtrating deep-sea sponges meet a unique upraised large-bodied assemblage of bathyal species on the Yermak Plateau.
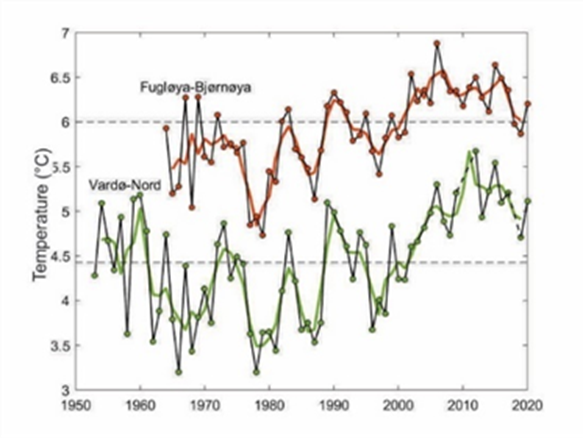
Figure CC.1: Average temperature at 50-200 m depth in the western Fugløya-Bear Island transect and the eastern Vardø-North section transect. Black lines show annual August-September values and thick lines show three-year running means. Horizontal lines show average temperature between 1981-2010. Graph from ICES WGIBAR, 2021
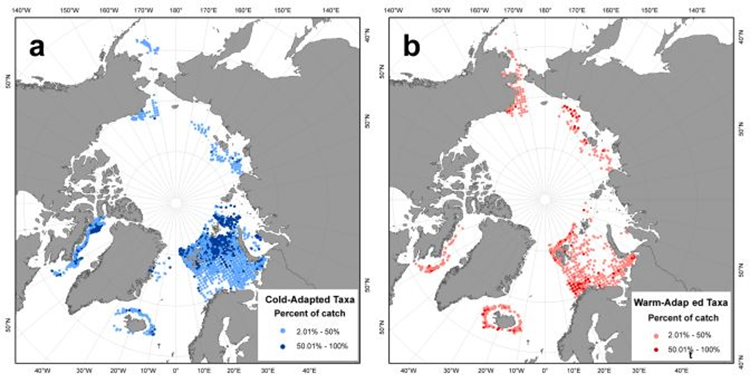
Figure CC.2. Distribution of (a) cold-water and (b) warm-water taxa, as percentage of catch (greater and less than 50% of catch) within each grid cell (50 km × 50 km). Note: Canadian taxa data from shrimp surveys were removed because species identification was limited until recently. From: Jørgensen et al., 2022a
Impacts on threatened and declining habitats and benthic species:
A rapid evaluation using the Marine Evidence-based Sensitivity Assessment (MarESA; https://www.marlin.ac.uk/habitats/biotopes) was undertaken to summarise the main sensitivities to climate change of the OSPAR threatened and / or declining habitats. This was done by considering the ‘high’ sensitivity classification in MarESA for the following climate change-related variables (Table CC.1):
The criteria and approach for the use of the different scenarios (high, middle, extreme, local) can be found here: https://www.marlin.ac.uk/sensitivity/SNCB-benchmarks
Table CC.1: Climate change pressure benchmarks derived from MarLIN MarESA, (please see for full detail: https://marlin.ac.uk/sensitivity/SNCB-benchmarks)
MarESA Pressure Assessment Benchmark | Definition |
Global warming (high, middle) | Middle emission scenario benchmark (by the end of this century (2081-2100): A 3°C rise in SST, NBT (coastal to the shelf seas) and surface air temperature (in eulittoral and supralittoral habitats). A 1°C rise in deep-sea habitats (>200 m) off the continental shelf. A 2°C rise in surface air temperature in intertidal habitats exclusive to Scotland. High emission scenario benchmark by the end of this century (2081-2100): A 4°C rise in SST, NBT (coastal to the shelf seas) and surface air temperature (in eulittoral and supralittoral habitats). A 1°C rise in deep-sea habitats (>200 m) off the continental shelf, and A 3°C rise in surface air temperature in intertidal habitats exclusive to Scotland. |
Marine heatwaves (high, middle) | Middle emission scenario benchmark: A marine heatwave occurring every three years, with a mean duration of 80 days, with a maximum intensity of 2°C. High emission scenario benchmark: A marine heatwave occurring every two years, with a mean duration of 120 days, and a maximum intensity of 3.5°C. |
Ocean acidification (high, middle) | Middle emission scenario benchmark: A further decrease in pH of 0.15 (annual mean) and corresponding 35% increase in H+ ions with no coastal aragonite undersaturation and the aragonite saturation horizon in the NE Atlantic, off the continental shelf, at a depth of 800 m by the end of this century (2081-2100). High emission scenario benchmark: A further decrease in pH of 0.35 (annual mean) and corresponding 120% increase in H+ ions, seasonal aragonite saturation of 20% of UK coastal waters and North Sea bottom waters, and the aragonite saturation horizon in the NE Atlantic, off the continental shelf, occurring at a depth of 400 m by the end of this century (2081-2100). |
Sea level rise (extreme, high, middle) | Middle emission scenario benchmark: A 50 cm rise in average UK sea-level rise by the end of this century (2081-2100). High emission scenario benchmark: A 70 cm rise in average UK by the end of this century (2081-2100). Extreme scenario benchmark: A 107 cm rise in average UK by the end of this century (2081-2100). |
Temperature increase (local) | An increase in 5°C for one month or 2°C for one year. |
Temperature decrease (local) | A decrease in 5°C for one month or 2°C for one year. |
Salinity increase (local) | An increase in one MNCR salinity category outside the usual range of the biotope/habitat for one year. Please see MarLIN MarESA for salinity category definitions: https://marlin.ac.uk/glossarydefinition/salinity |
Salinity decrease (local) | A decrease in one MNCR salinity category outside the usual range of the biotope/habitat for one year. Please see MarLIN MarESA for salinity category definitions: https://marlin.ac.uk/glossarydefinition/salinity |
Water flow (tidal current) changes (local) | A change in peak mean spring bed flow velocity of between 0.1m/s to 0.2m/s for more than 1 year. |
Emergence regime changes (A change in the time covered or not covered by the sea for a period of => 1 year | A change in the period covered or not covered by the sea for a period of ≥ 1 year. Or An increase in relative sea level or decrease in high water level for ≥ 1 year. |
Wave exposure changes (local) | A change in nearshore significant wave height >3% but <5% for one year. |
- Global warming (high, middle);
- Marine heatwaves (high, middle);
- Ocean acidification (high, middle);
- Sea level rise (extreme, high and middle);
- Temperature increase (local)
- Temperature decrease (local);
- Salinity increase (local);
- Salinity decrease (local);
- Water flow (tidal current) changes (local);
- Emergence regime changes (A change in the period covered or not covered by the sea for a period of ≥ 1 year);
- Wave exposure changes (local).
Carbonate mounds, Haploops habitat and Cymodocea meadows were excluded due to the lack of information available within the MarESA sources. (https://www.marlin.ac.uk/sensitivity/sensitivity_rationale).
Global warming and marine heatwave pressures were assessed to be causing the largest impacts on the majority of communities comprising kelp forest, maerl bed and Modiolus modiolus bed habitats. Sea-level rise was also listed as highly impactful for kelp forest. Ocean acidification was assessed as likely to represent a serious threat to coral gardens, Lophelia pertusa reefs, and maerl beds.
Climate change can have a compounding effect on threats already impacting habitats. For example, for Zostera beds, existing threats such as increased water turbidity / reduced light penetration and sediment re-suspension may be exacerbated by climate change in the future, and climate change may also increase the need to further reduce other stressors in order to facilitate expansion of Zostera beds to deeper, cooler waters (Krause-Jensen et al., 2021).
Changes in coastal development due to increased needs for coastal protection as a result of climate change impacts could lead to increased risks for habitat loss and increased risks of coastal squeeze, i.e. the coastal zone in built-up areas becoming narrower due to a rising sea level. This may also need to be taken into consideration to ensure that future management becomes “climate ready” to protect, for example, Zostera beds.
Local salinity changes (i.e., increase or decrease) were found to have a significant impact on coral gardens, deep-sea sponge aggregations, kelp forests, littoral chalk communities, Lophelia pertusa reefs, Modiolus modiolus beds and sea-pen and burrowing megafauna communities. Water flow (tidal current) changes were likely to impact maerl beds and sea-pen and burrowing megafauna communities. Littoral chalk communities were also found to be highly affected by emerging regime changes.
Intertidal mudflats can be affected by climate-change induced sea-level rise and increased storm frequency, affecting the sedimentation patterns of mudflats and estuaries, coastal squeeze (e.g., in the United Kingdom) and changes in exposure time. Climate change also leads to temperature increase resulting in changing ecological interactions between species.
The predictions of future trends (2020-2030) for the condition of deep-sea sponge aggregations are quite challenging. Modelling predicts shifts in temperature, pH, oxygen, and particulate organic carbon (i.e., food supply) in the bathyal zone (i.e. the zone where several deep-sea sponge aggregations have been found) for OSPAR Regions by 2100 under Representative Concentration Pathway (RCP)8.5. By combining (i) the model predictions for abiotic / biotic conditions in bathyal habitats (see above) and (ii) the scientific knowledge about the impacts of temperature, acidification and diet / feeding metabolism, it is possible to suggest that future environmental conditions in the OSPAR Maritime Area may contribute to deterioration in the condition of deep-sea sponge aggregations. However, further research is needed to advance scientific knowledge on this front. The northernmost regions of the North Atlantic are predicted to experience the greatest impacts under worst-case scenario predictions. Increased temperature and lower pH will drastically reduce the suitable habitat for ecosystem-engineer species and lead to declines in population densities, loss of biodiversity and reduced biogeographic distribution that might compromise large-scale connectivity and long-term survival. The reductions in carbon fluxes in oligotrophic areas may be even more serious. The predicted effects of climate change on deep-sea sponge aggregations by 2100 are changes in distribution, extent and condition. Changes in circulation due to the slowdown of the Atlantic Meridional Overturning Circulation (which affects all OSPAR Regions) will influence regional and local circulation patterns, which will also alter patterns of population connectivity.
Climate change threatens to induce significant shifts in the biological communities on carbonate mounds in the Wider Atlantic and the impact processes that support these features, through altered hydrodynamic regimes, a re-distribution of primary productivity and the availability of organic matter on the seafloor, and ocean acidification. Regarding the latter, the ICES Report on Ocean Climate Change, 2014 (Larsen, et al., 2016) has predicted that under RCP4.5, the waters surrounding the Hatton and Porcupine Banks will be approaching aragonite undersaturation by 2100. Climate change and ocean acidification impacts on carbonate mounds remain unknown, even after the last assessment, and either may not yet have occurred or may not have been measured in situ on these listed features. However, expert judgement suggests that these impacts could have started to take place, as even short-term exposure to decreased pH can impact cold-water corals, and these pressures are likely to increase over the next 6 to 12 years.
There was no explicit implementation reporting in 2019 on climate change under paragraph 3.1b of OSPAR Recommendation 2014/10: “assessing whether existing management measures for the protection of carbonate mounds are effective, and determine whether further measures are needed to address the key threats, including the potential impacts from climate change and ocean acidification”.
Although ocean acidification is clearly continuing, the current and immediate future impacts on coral gardens are difficult to quantify.
Deep-sea corals, especially those with aragonite rather than calcite skeletons, are vulnerable to the effects of ocean acidification, with impacts predicted by the end of this century. The ICES Report on Ocean Climate Change, 2014 (Larsen, et al., 2016) predicts that, under RCP4.5, the waters surrounding the Reykjanes Ridge, the southern Iceland shelf and the edge of the Hatton and Porcupine Banks will be approaching aragonite undersaturation by 2100. Under RCP8.5 most of the North Atlantic will be undersaturated except for the Celtic margin and the Bay of Biscay. Other models of aragonite saturation states under RCP8.5 have predicted similar results (e.g., Puerta et al., 2020), including for specific coral garden taxa such as Acanella and Paragorgia (Morato et al., 2020). Predictions tend not to take into account the potential increased vulnerability of early life stages to ocean acidification.
Other effects of climate change likely to impact coral gardens by the end of the century include increases in temperature affecting distribution, decreased particulate organic carbon inputs, and the slowing of the Atlantic Meridional Overturning Circulation, which could have broad-scale impacts on the whole of the North Atlantic.
It has been shown that meridional overturning circulation has the potential to increase acidification in the deep Atlantic Ocean, with rapid shoaling of the aragonite saturation horizon, particularly in the subpolar North Atlantic, possible within the next three decades (Perez et al., 2018). Acidification can impact the integrity of coral reefs and cause ecosystem-scale habitat loss (Hennige et al., 2020). Some recent studies, however, suggest that Lophelia may contain sufficient genetic variability to adapt to future ocean acidification (Kurman et al., 2017), at least in some regions (Georgian et al., 2016).
Morato et al., (2020) used habitat suitability modelling to compare present-day (1951–2000) and projected Lophelia distributions under future (2081–2100; RCP8.5) environmental conditions. The models predict a decrease of approximately 80% in suitable habitat for Lophelia pertusa by 2100 due to a combination of factors. The authors stress the importance of identifying and preserving species climate refugia when planning conservation measures, to improve resilience against climate change.
Although adult vent organisms are adapted to oscillations and variable low pH, high temperature and low oxygen in vent fluids, it is not known whether species are adapted to permanent change in environmental variables. To what degree, and whether, vent larvae that disperse via ocean currents are adapted to changes in pH, temperature and oxygen in the ocean, is unknown. Changes induced by climate stressors, such as altered circulation due to the slowdown of the Atlantic Meridional Overturning Circulation (AMOC), can impact connectivity at regional scales and may even increase the risk of species extinction, especially given the fragmented nature of these environments and the planktonic nature of many vent species.
Overarching impacts on benthic habitats from climatic drivers and ocean acidification:
Table CC.2 is a high-level evaluation of the evidence available on the impacts of climatic drivers and ocean acidification on broad-scale habitats.
Table CC.2 : Overarching foreseen impacts (experts’ judgement) on North-East Atlantic benthic habitats, from climatic drivers and ocean acidification. (Link to full table)
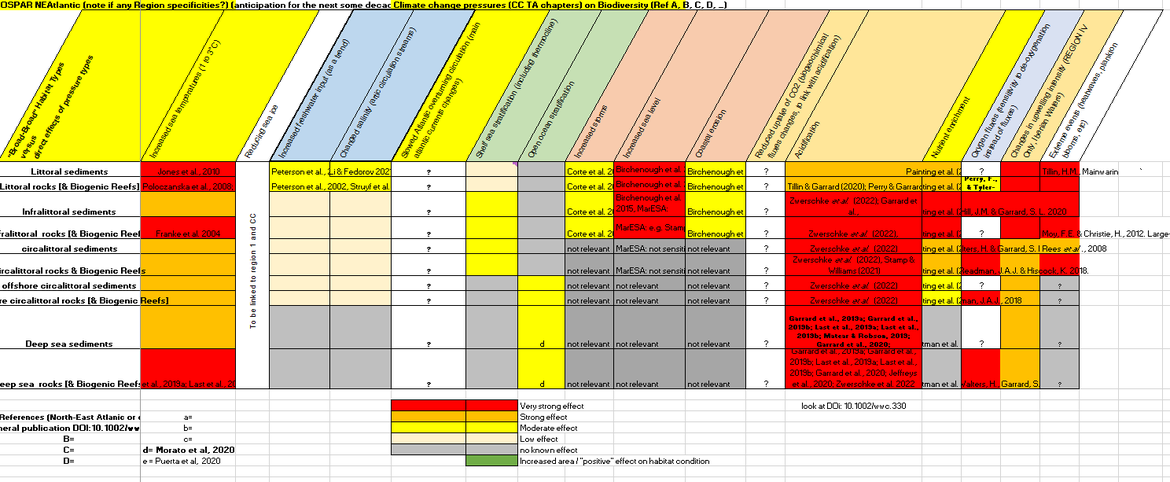
An overview of climate change-related impacts on the marine benthos in the North Atlantic is published in Birchenough et al., (2015), which tackles the relationship between different physical aspects of climate change and the marine benthos and covers: (a) the responses to changes in seawater temperature (biogeographic shifts and phenology); (b) altered Hydrodynamics; (c) ocean acidification (OA); and (d) sea-level rise coastal squeeze.
Ocean acidification
Ocean acidification is the reduction in the pH of the ocean caused by uptake of excess carbon dioxide (CO2) from the atmosphere. This is a natural process, but the accelerated release of anthropogenically produced CO2 into the atmosphere is happening too fast for the natural system to keep pace. The effects of ocean acidification on marine benthic species and habitats are not well understood. Marine species with calcium carbonate shells and skeletons, such as corals and mussels, are particularly vulnerable. Often species are exposed and affected by ocean acidification across their life cycle, including their early development and larval stages. Thus, ocean acidification may not only impact established populations, but also reduce the likelihood of successful reproduction to maintain these populations. Understanding the extent and magnitude of these impacts is important because they could result in reductions in sensitive habitats and species and continued declines in biodiversity. This can have knock-on effects on the food web (e.g., prey availability) and the ecosystem services provided (e.g., commercial fishing, carbon storage). An initial study was undertaken to evaluate and identify seabed species and habitats and their associated larval stages most sensitive to ocean acidification, and to identify the areas most likely to experience the largest changes in pH. The results showed that, regardless of the emissions scenario, significant changes in ocean pH and carbonate chemistry alongside changes in benthic communities can be expected within the next three decades. These changes are likely to be more pronounced in certain areas such as the northern North Sea, the eastern Channel and the Irish Sea (Zwerschke et al., 2022).
Increased sea temperature
Species can adapt to increases in temperature by changing their depth and / or latitude distribution ranges. Species able to change both ranges (depth and latitude) will be less sensitive than species which cannot modify their depth range. Because of this it is considered that algae communities (restricted to depth ranges with enough light availability) will be more threatened by the increase of temperatures than invertebrate communities living in sediment habitats. In the same way, cold-water corals living near the aragonite saturation horizon will also be more threatened than species living far from this horizon.
Increased freshwater inputs and changed salinity
The impacts of changes in salinity are very region-dependent. For example, in the Baltic Sea, salinity is a major driver of diversity, which is not the case for open-water regions such as the Bay of Biscay and Iberian Coast (OSPAR Region IV).
Climate change can result in changes to the timing and extent (relative importance) of freshwater inputs, with (sub)regional differences in precipitation resulting in either increased or decreased freshwater inputs.
In general, it is thought that both changed salinity and changed freshwater inputs will have low direct impact on benthic communities, although they can be locally important in littoral communities, especially near river mouths.
Slowed Atlantic Meridional Overturning Circulation (AMOC)
The slowing of the AMOC will have strong impacts on the climate of Europe. Although it is not possible to forecast the exact impacts on specific benthic communities, the expected change is likely to be considerable. Currents influence sediment types, which have a major impact on benthic communities and are also key for filter feeding organisms such as corals and sponges. However, without an understanding of how these changes will occur specifically, it is not possible to forecast their impacts on the benthos, which need not necessarily be exclusively negative.
Nutrient enrichment
The potential impacts of climate change include changes in the magnitude of nutrient enrichment in estuarine, coastal and offshore waters, in physical and chemical oceanography (e.g., circulation patterns, acidification and light) and in the rates of biogeochemical cycling in these waters. Nutrient enrichment leads to a number of negative impacts on benthic habitats, such as community changes (see: Condition of Benthic Habitat Communities: Assessment of some Coastal Habitats in Relation to Nutrient and/or Organic Enrichment (BH2a) , Drivers , Activities , Pressures ). Climate effects are typically related to changes in precipitation and peaks / extremes in run-off, including nutrient loads. It is difficult to distinguish between anthropogenic and climate change-driven nutrient effects (related processes). Typically, the largest effects have been observed in river plumes and shallow areas, due to the increase in algal blooms there which change light conditions and species compositions, potentially also leading to oxygen depletion (Painting et al., 2013). It is also expected that increased temperatures may decrease denitrification. Higher concentrations of nitrate may lead to a switch to phosphate as the limiting nutrient. Increased storminess would increase nutrient concentrations at the ocean surface and may increase supply to shelf seas like the North Sea. Modelling of ocean productivity in a warmer climate suggests that increased stratification in summer will limit nutrient supply to surface waters during the productive seasons and inhibit mixing due to storms in winter. The most likely effects on shelf seas are largely unclear at the moment (Mills and Hydes, 2006). With regard to deep-sea habitats, increased stratification and therefore limited transport to the seafloor might counteract or even overrule increased nutrient input and, more likely, lead to nutrient decreases in deep-sea benthic habitats. The resulting impact on benthic community levels is largely unclear (Sweetman et al., 2017).
Increased sea level and changes to storms and coastal erosion
Sea-level rise increases water depth at the shore and results in changes in wave and tidal energy along the coast (Wolf et al., 2020). Benthic habitats such as subtidal bedforms, intertidal flats, saltmarshes and sand dunes migrate along and parallel to the shore to maintain their position in the coastal energy gradient (Garrard and Tyler-Walters, 2020). Sedimentary habitats are liable to adapt to a gradual sea level rise and compensate for alterations by extra sedimentation. However, coastal habitats and particularly tidal flats may suffer substantial losses if sea level increases too rapidly and where coastal defence structures prevent their natural movement (coastal squeeze) (Birchenough et al., 2015; Garrard and Tyler-Walters, 2020). Infralittoral and intertidal rock and biogenic reef habitats (e.g., blue mussel beds, honeycomb worm reefs) that are unable to migrate landwards are particularly threatened by increasing water depths (Stamp and Williams, 2021; Tillin et al., 2020). Large areas of macrophyte-dominated habitats such as seagrass beds and kelp forests may also be lost, as an increase in depth reduces the light available to the plants (d’Avack et al., 2022a; d’Avack et al., 2022b; Stamp et al., 2020).
Human interventions to mitigate coastal erosion may add to the ecological impact of sea-level rise (Birchenough et al., 2015). In particular, ‘hard’ coastal protection structures such as dykes can alter hydrological and sediment dynamics along the shoreline and thereby also affect benthic biodiversity and productivity (Spreybroeck et al., 2006). The effects of sea-level rise may be further increased by storms and storm surges, which in turn will raise the need for coastal protection measures. Higher wave energy during storms may translocate and disperse large sediment volumes, thus altering sediment properties and reducing habitat heterogeneity. While the majority of the species occurring in coastal habitats are adapted to high-energy environments and may recover within a short time frame, the impact on benthic habitats will increase with the magnitude, frequency, and spatial scale of storm events (Corte et al., 2017).
Extreme events (heatwaves)
Marine heatwaves are increasingly becoming a global concern, with impacts from warm-water events predicted to increase in frequency in the coming years. These are defined as occurring when the surface temperature exceeds a threshold greater than five consecutive days (Brauko et al., 2020). These prolonged events are associated with coral bleaching, reductions in kelp forests and seagrass meadows (Holbrook et al., 2020), reduced surface chlorophyll (Bond et al., 2015), loss of invertebrates (Caputi et al., 2016), species range shifts coupled with community restructuring (Cavole et al., 2016; Wernberg et al., 2016), alteration of fishery quota and closures (Cavole et al., 2016) and tensions between nations. Warm-water events can vary with extent and depth and are dependent on the geometry of regions and the mechanisms that cause and maintain them (Reed et al., 2016). In addition to a long-lasting global increase in marine temperatures, discrete extreme warm-water events are projected to become more frequent, intense, and longer lasting in the 21st century (Meehl and Tebaldi, 2004). The 2012 event in the North-West Atlantic saw significant shifts in geographical and seasonal shifts in several marine species, leading to altered fishing activities and harvesting patterns and substantial political and economic complications for the region (Mills et al., 2013). Further study is needed to improve knowledge of the impacts from marine heatwaves on benthic habitats in the North-East Atlantic.
Further work to improve assessment of climate change effects on benthic habitats
Benthic indicators have been developed to measure the pressures caused by human impacts (e.g., trawling disturbance, eutrophication) on benthic habitats across regions. They take into account relevant environmental parameters such as depth in order to calculate the different degrees of impact. However, the current design is not sufficiently sensitive to measure changes driven by climatic factors and / or ocean acidification. To fill the gaps, new or adapted indicators that include additional environmental parameters might be needed to measure the impact of climate change on benthic habitats. Although currently no specific indicators have been developed for this task in the framework of OSPAR’s Benthic Habitats Expert Group (OBHEG), the scientific literature contains good examples that could be adapted. For instance, it will be possible to predict the impact of climate change in the habitat of different relevant species by OSPAR Region, measuring the loss or reduction of suitable habitat as a consequence of climate change (Morato et al., 2020). On the other hand, use can be made of metrics such as Preferred Temperature of the Community (PTC), which is calculated by weighting species’ preferred temperature by their annual abundance (Collie et al., 2008). This has already been used in the Bay of Biscay and the Iberian Coast Region to measure the impact of climate change on fish communities (Punzón et al., 2021). Another example is the Intertidal Community Temperature Index, which measures the status of a benthic community in terms of the composition of cold- and warm-water species. It is quantitative, easily applied, and gives a direct measurement of the response to climate and climate change across all the species in a community. This information is essential for understanding how anthropogenic pressure exacerbates the effects of climate change (Burrows et al., 2018).
Birchenough, S.N.R., Reiss, H., Degraer, S., Mieszkowska, N., Borja, A., Buhl-Mortensen, L., Braeckman, U., Craeymeersch, J., De Mesel, I., Kerckhof, F., Kröncke, I., Parra, S., Rabaut, M., Schröder, A., Van Colen, C., Van Hoey, G., Vincx, M., Wätjen, K. (2015) ‘Climate Change and marine benthos: a review of existing research and future directions in the North Atlantic’, Wiley interdisciplinary reviews: Climate Change 03/2015; 6(2):6: 203-223. Available at: https://doi.org/10.1002/wcc.330
Bond, N.A., Cronin, M.F., Freeland, H. and Mantua, N. (2015) ‘Causes and impacts of the 2014 warm anomaly in the NE Pacific’, Geophysical Research Letters, 42(9), pp. 3414–3420. Available at: https://doi.org/10.1002/2015GL063306
Brauko, K.M. Cabra, l A., Costa, N.V., Hayden, J., Dias, C.E.P., Leite, E.S., Westphal, R.D., Mueller, C.M., Hall-Spencer, J.M., Rodrigues, R.R., Rörig LR, Pagliosa PR, Fonseca, A.L., Alarcon, O.E. and Horta, P.A. (2020) ‘Marine Heatwaves, Sewage and Eutrophication Combine to Trigger Deoxygenation and Biodiversity Loss: A SW Atlantic Case Study’, Frontiers in Marine Science, 0. Available at: https://doi.org/10.3389/fmars.2020.590258
Buhl-Mortensen, P., Dolan, M.F.J., Ross, R.E., Gonzalez-Mirelis, G., Buhl-Mortensen, L., Bjarnadóttir, L.R. and Albretsen, J. (2020) ‘Classification and Mapping of Benthic Biotopes in Arctic and Sub-Arctic Norwegian Waters’, Frontiers in Marine Science 7, p. 271. Available at: https://doi.org/10.3389/fmars.2020.00271
Burrows M.T, , Mieszkowska, N., Baxter, J., Vina-Herbon, C., Singleton, G., Robison, K. and Young, M. 2018. Intertidal community index (MarClim). UK Marine Online Assessment Tool, available at: https://moat.cefas.co.uk/biodiversity-food-webs-and-marine-protected-areas/benthic-habitats/intertidal-community-index/
Caputi, N., Kangas, M., Denham, A., Feng, M., Pearce, A., Hetzel, Y. and Chandrapavan, A. (2016) ‘Management adaptation of invertebrate fisheries to an extreme marine heat wave event at a global warming hot spot’, Ecology and Evolution, 6(11), pp. 3583–3593. Available at: https://doi.org/10.1002/ece3.2137
Cavole, L.M., Demko, A.M., Diner, R.E., Giddings, A., Koester, I., Pagniello, C.M.L.S., Paulsen, M.-L., Ramirez-Valdez, A., Schwenck, S.M., Yen, N.K., Zill, M.E. and Franks, P.J.S. (2016) ‘Biological impacts of the 2013–2015 warm-water anomaly in the Northeast Pacific: winners, losers, and the future’, Oceanography, 29(2), pp. 273–285.
Collie, J.S., Wood, A.D. and Jeffries, H.P. 2008. Long-term shifts in the species composition of a coastal fish community. Canadian Journal of Fisheries and Aquatic Sciences, 65(7), pp.1352-1365
Corte, G. N., Schlacher, T. A., Checon, H. H., Barboza, C. A., Siegle, E., Coleman, R. A., and Amaral, A. C. Z. (2017) ‘Storm effects on intertidal invertebrates: increased beta diversity of few individuals and species’, PeerJ, 5, e3360. Available at: https://peerj.com/articles/3360/
D'Avack, E.A.S., Tyler-Walters, H., Wilding, C.M. and Garrard, S.L. (2022a) ‘Zostera (Zosterella) noltei beds in littoral muddy sand’, In Tyler-Walters H. and Hiscock K. (eds) Marine Life Information Network: Biology and Sensitivity Key Information Reviews, [on-line]. Plymouth: Marine Biological Association of the United Kingdom. Available at: https://www.marlin.ac.uk/habitats/detail/318
D'Avack, E.A.S., Tyler-Walters, H., Wilding, C.M. and Garrard, S.L. (2022b.) ‘Zostera (Zostera) marina beds on lower shore or infralittoral clean or muddy sand’, In Tyler-Walters H. and Hiscock K. (eds) Marine Life Information Network: Biology and Sensitivity Key Information Reviews, [on-line]. Plymouth: Marine Biological Association of the United Kingdom. Available at: https://www.marlin.ac.uk/habitats/detail/257
Garrard, S. L., and Tyler-Walters, H. (2020) ‘Habitat (biotope) sensitivity assessments for climate change pressures’, Report from the Marine Life Information Network (MarLIN), to Dept. for Environment, Food and Rural Affairs (Defra) & Joint Nature Conservation Committee (JNCC). Marine Biological Association of the United Kingdom, Plymouth. Available at: https://www.marlin.ac.uk/assets/pdf/Climate-change-pressures-Feb2020.pdf
Gattuso, J.P., Magnan, A., Billé, R., Cheung, W.W., Howes, E.L., Joos, F., Allemand, D., Bopp, L., Cooley, S.R., Eakin, C.M. and Hoegh-Guldberg, O. (2015) ‘Contrasting futures for ocean and society from different anthropogenic CO2 emissions scenarios’, Science, 349(6243), p.aac4722. Available at: https://www.science.org/doi/10.1126/science.aac4722
Georgian, S.E., Dupont, S., Kurman, M., Butler, A., Strömberg, S.M., Larsson, A.I. and Cordes, E.E. (2016) ‘Biogeographic variability in the physiological response of the cold‐water coral L ophelia pertusa to ocean acidification’, Marine Ecology, 37(6), pp.1345-1359. Available at: https://doi.org/10.1111/maec.12373
Harley, C.D., Randall Hughes, A., Hultgren, K.M., Miner, B.G., Sorte, C.J., Thornber, C.S., Rodriguez, L.F., Tomanek, L. and Williams, S.L. (2006) ‘The impacts of climate change in coastal marine systems’, Ecology letters, 9(2), pp.228-241. Available at: https://onlinelibrary.wiley.com/doi/epdf/10.1111/j.1461-0248.2005.00871.x
Hennige, S.J., Wolfram, U., Wickes, L., Murray, F., Roberts, J.M., Kamenos, N.A., Schofield, S., Groetsch, A., Spiesz, E.M., Aubin-Tam, M.E. and Etnoyer, P.J. (2020) ‘Crumbling reefs and cold-water coral habitat loss in a future ocean: evidence of “Coralporosis” as an indicator of habitat integrity’, Frontiers in Marine Science, p.668. Available at: https://doi.org/10.3389/fmars.2020.00668
Hoegh-Guldberg, O. and Bruno, J.F. (2010) ‘The impact of climate change on the world’s marine ecosystems’, Science, 328(5985), pp.1523-1528. Available at: https://www.science.org/doi/10.1126/science.1189930
Holbrook, N.J., Sen Gupta, A., Oliver, E.C.J., Hobday, A.J., Benthuysen, J.A., Scannell, H.A., Smale, D.A. and Wernberg, T. (2020) ‘Keeping pace with marine heatwaves’, Nature Reviews Earth & Environment, 1(9), pp. 482–493. Available at: https://doi.org/10.1038/s43017-020-0068-4
ICES (2021), ‘Working Group on the Integrated Assessments of the Barents Sea (WGIBAR)’, ICES Scientific Reports. Available at: https://doi.org/10.17895/ices.pub.8241
Jorgensen, C.F., Powell, L.A., Lusk, J.J., Bishop, A.A., Fontaine, J.J. (2014) ‘Assessing Landscape Constraints on Species Abundance: Does the Neighborhood Limit Species Response to Local Habitat Conservation Programs?’, PLoS ONE 9(6): e99339. Available at: https://doi.org/10.1371/journal.pone.0099339
Jørgensen, L.L., Logerwell, E.A., Strelkova, N., Zakharov, D., Roy, V., Nozeres, C., Bluhm, B.A., Ólafsdóttir, S.H., Burgos, J.M., Sørensen, J. and Zimina, O. (2022a) ‘International megabenthic long-term monitoring of a changing arctic ecosystem: Baseline results’, Progress in Oceanography, 200, p.102712. Available at: https://doi.org/10.1016/j.pocean.2021.102712
Jørgensen, L.L., Pecuchet, L., Ingvaldsen, R.B. and Primicerio, R. (2022b) ‘Benthic transition zones in the Atlantic gateway to a changing Arctic ocean’, Progress in Oceanography, 204, p.102792. Available at: https://doi.org/10.1016/j.pocean.2022.102792
Krause-Jensen, D., Duarte, C.M., Sand-Jensen, K. and Carstensen, J. (2021) ‚Century-long records reveal shifting challenges to seagrass recovery’, Global Change Biology 27: 563–575. Available at: https://doi.org/10.1111/gcb.15440
Kurman, M.D., Gomez, C.E., Georgian, S.E., Lunden, J.J. and Cordes, E.E. (2017) ‘Intra-specific variation reveals potential for adaptation to ocean acidification in a cold-water coral from the Gulf of Mexico’, Frontiers in Marine Science, 4, p.111. Available at: https://doi.org/10.3389/fmars.2017.00111
Larsen, Karin M. H., Gonzalez-Pola, C., Fratantoni, P., Möller, A.B., Hughes, S. L. (2016) ‘ICES Report on Ocean Climate 2014’, ICES Cooperative Research Reports (CRR). Available at: https://doi.org/10.17895/ices.pub.5136
Meehl, G.A. and Tebaldi, C. (2004) ‘More Intense, More Frequent, and Longer Lasting Heat Waves in the 21st Century’, Science, 305(5686), pp. 994–997. Available at: https://doi.org/10.1126/science.1098704
Mills, D. and Hydes, D. (2006). Impacts of Climate Change on Nutrient Enrichment in Marine Climate Change Impacts Annual Report Card 2006 (Eds. Buckley, P.J, Dye, S.R. and Baxter, J.M), Online Summary Reports, MCCIP, Lowestoft, www.mccip.org.uk
Mills, K.E., Pershing, A.J., Brown, C.J., Chen, Y., Chiang, F.-S., Holland, D.S., Lehuta, S., Nye, J.A., Sun, J.C., Thomas, A.C. and Wahle, R.A.(2013) ‘Fisheries management in a changing climate: lessons from the 2012 ocean heat wave in the Northwest Atlantic’, Oceanography, 26(2), pp. 191–195.
Morato, T., González‐Irusta, J.M., Dominguez‐Carrió, C., Wei, C.L., Davies, A., Sweetman, A.K., Taranto, G.H., Beazley, L., García‐Alegre, A., Grehan, A. and Laffargue, P. (2020) ‘Climate‐induced changes in the suitable habitat of cold‐water corals and commercially important deep‐sea fishes in the North Atlantic’, Global Change Biology, 26(4), pp.2181-2202. Available at: https://doi.org/10.1111/gcb.14996
Nagelkerken, I. and Connell, S.D. (2015) ‘Global alteration of ocean ecosystem functioning due to increasing human CO2 emissions’, Proceedings of the National Academy of Sciences, 112(43), pp.13272-13277. Available at: https://www.pnas.org/doi/full/10.1073/pnas.1510856112
Painting, S., Foden, J., Forster, R., Van der Molen, J., Aldridge, J., Best, M., Jonas, P., Walsham, P., Webster, L., Gubbins, M., Heath, M., McGovern, E., Vincent, C., Gowen, R. and O’Boyle, S. (2013). Impacts of climate change on nutrient enrichment, MCCIP Science Review 2013, 219-235, https://doi.org/10.14465/2013.arc23.219-235
Perez, F.F., Fontela, M., García-Ibáñez, M.I., Mercier, H., Velo, A., Lherminier, P., Zunino, P., de La Paz, M., Alonso-Pérez, F., Guallart, E.F. and Padin, X.A. (2018) ‘Meridional overturning circulation conveys fast acidification to the deep Atlantic Ocean’, Nature, 554(7693), pp.515-518. Available at: https://doi.org/10.1038/nature25493
Poloczanska, E.S., Brown, C.J., Sydeman, W.J., Kiessling, W., Schoeman, D.S., Moore, P.J., Brander, K., Bruno, J.F., Buckley, L.B., Burrows, M.T. and Duarte, C.M. (2013) ‘Global imprint of climate change on marine life’, Nature Climate Change, 3(10), pp.919-925. Available at: https://doi.org/10.1038/nclimate1958
Poloczanska, E.S., Burrows, M.T., Brown, C.J., García Molinos, J., Halpern, B.S., Hoegh-Guldberg, O., Kappel, C.V., Moore, P.J., Richardson, A.J., Schoeman, D.S. and Sydeman, W.J. (2016) ‘Responses of marine organisms to climate change across oceans’, Frontiers in Marine Science, p.62. Available at: https://doi.org/10.3389/fmars.2016.00062
Puerta, P., Johnson, C., Carreiro-Silva, M., Henry, L.A., Kenchington, E., Morato, T., Kazanidis, G., Rueda, J.L., Urra, J., Ross, S. and Wei, C.L. (2020) ‘Influence of water masses on the biodiversity and biogeography of deep-sea benthic ecosystems in the North Atlantic’, Frontiers in Marine Science, p.239. Available at: https://doi.org/10.3389/fmars.2020.00239
Punzón, A., López-López, L., González-Irusta, J.M., Preciado, I., Hidalgo, M., Serrano, A., Tel, E., Somavilla, R., Polo, J., Blanco, M. and Ruiz-Pico, S. 2021. Tracking the effect of temperature in marine demersal fish communities. Ecological Indicators, 121, p.107142.
Reed, D. Washburn, L., Rassweiler, A., Miller, R., Bell, T. and Harrer, S. (2016) ‘Extreme warming challenges sentinel status of kelp forests as indicators of climate change’, Nature Communications, 7(1), p. 13757. Available at: https://doi.org/10.1038/ncomms13757
Skagseth, Ø., Eldevik, T., Årthun, M., Asbjørnsen, H., Lien, V.S. and Smedsrud, L.H. (2020) ‘Reduced efficiency of the Barents Sea cooling machine’, Nature Climate Change, 10(7), pp.661-666. Available at: https://doi.org/10.1038/s41558-020-0772-6
Speybroeck, J., Bonte, D., Courtens, W., Gheskiere, T., Grootaert, P., Maelfait, J.-P., Mathys, M., Provoost, S., Sabbe, K., Stienen, E.W.M., Van Lancker, V., Vincx, M., Degraer, S. (2006) ‘Beach nourishment: an ecologically sound coastal defence alternative? A review’, Aquatic conservation: Marine and Freshwater ecosystems 16.4: 419-435. Available at: https://doi.org/10.1002/aqc.733
Stamp, T.E. and Williams, E. (2021) ‘Alaria esculenta, Mytilus edulis and coralline crusts on very exposed sublittoral fringe bedrock’, In Tyler-Walters H. and Hiscock K. (eds) Marine Life Information Network: Biology and Sensitivity Key Information Reviews, [on-line]. Plymouth: Marine Biological Association of the United Kingdom. Available at: https://www.marlin.ac.uk/habitats/detail/217
Stamp, T.E., Hiscock, K. and Garrard, S. L. (2020) ‘Laminaria hyperborea forest with a faunal cushion (sponges and polyclinids) and foliose red seaweeds on very exposed upper infralittoral rock’, In Tyler-Walters H. and Hiscock K. (eds) Marine Life Information Network: Biology and Sensitivity Key Information Reviews, [on-line]. Plymouth: Marine Biological Association of the United Kingdom. Available at: https://www.marlin.ac.uk/habitats/detail/44
Sweetman, A.K., Thurber, A.R., Smith, C.R., Levin, L.A., Mora, C., Wei, C.-L., Gooday, A.J., Jones, D.O.B., Rex, M., Yasuhara, M., Ingels, J., Ruhl, H.A., Frieder, C.A., Danovaro, R., Würzberg, L., Baco, A., Grupe, B.M., Pasulka, A., Meyer, K.S., Dunlop, K.M., Henry, L.-A. and Roberts, J.M. (2017). Major impacts of climate change on deep-sea benthic ecosystems. Elementa Science of the Anthropocene, 5: 4, https://doi.org/10.1525/elementa.203
Tillin, H.M, Jackson, A. and Garrard, S. L. (2020) ‘Sabellaria alveolata reefs on sand-abraded eulittoral rock’, In Tyler-Walters H. and Hiscock K. (eds) Marine Life Information Network: Biology and Sensitivity Key Information Reviews, [on-line]. Plymouth: Marine Biological Association of the United Kingdom. Available at: https://www.marlin.ac.uk/habitats/detail/351
Weinert, M., Mathis, M., Kröncke, I., Neumann, H., Pohlmann, T. and Reiss, H. (2016) ‘Modelling climate change effects on benthos: Distributional shifts in the North Sea from 2001 to 2099’, Estuarine, Coastal and Shelf Science, 175, pp.157-168. Available at: https://doi.org/10.1016/j.ecss.2016.03.024
Wernberg, T., Wernberg, T., Bennett, S., Babcock, R.C., de Bettignies, T., Cure, K., Depczynski, M., Dufois, F., Fromont, J., Fulton, C.J., Hovey, R.K., Harvey, E.S., Holmes, T.H., Kendrick, G.A., Radford, B., Santana-Garcon, J., Saunders, B.J., Smale, D.A., Thomsen, M.S., Tuckett, C.A., Tuya, F., Vanderklift, M.A. and Wilson, S. (2016) ‘Climate-driven regime shift of a temperate marine ecosystem’, Science, 353(6295), pp. 169–172. Available at: https://doi.org/10.1126/science.aad8745
Weslawski, J.M., Wiktor, J., and Kotwicki, L. (2010) ‘Increase in biodiversity in the arctic rocky littoral, Sorkappland, Svalbard, after 20 years of climate warming’, Marine Biodiversity 40, pp. 123–130. Available at: https://doi.org/10.1007/s12526-010-0038-z
Wolf, J., Woolf, D. and Bricheno, L. (2020) Impacts of climate change on storms and waves relevant to the coastal and marine environment around the UK. MCCIP Science Review 2020, 132–157.
Zwerschke, N., McQuatters-Gollop, A., Hinchen, H., Pettit, L., Dinwoodie, K., Baigent, H. and Vina-Herbon, C. (2022) ‘Ocean acidification and the seabed: how do we detect early warning signals?’, Report ME5236 for the UK Department for the Environment, Food and Rural Affairs. 48 pp.
Cumulative Effects | Executive Summary and Five Questions |