Climate change and ocean acidification in pelagic habitats
All OSPAR Regions are currently experiencing an increase in sea surface temperature due to climate change and a decrease in pH due to ocean acidification. Elevated sea surface temperature has impacted seasonal stratification patterns, causing greater nutrient depletion in the growing season and shifting the base of pelagic food webs towards a less desirable state. Biodiversity indicators have revealed changes in sea surface temperature from 1960 to 2019 linked to widespread declines in the biomass and abundance of phytoplankton and zooplankton, particularly for shelf and oceanic / beyond shelf areas. Rising temperatures were linked to increasing trends in meroplankton abundance and decreasing trends in copepods throughout the Greater North Sea. Global efforts to slow climate change will probably be the best mechanism to counter widespread changes in plankton communities. Regionally targeted management measures are likely to generate noticeable impact only in coastal areas, but may have some effect in areas where plankton communities are affected by the cumulative impacts of multiple pressures (i.e., warming and eutrophication).
In the North-East Atlantic Ocean, sea surface temperature warming (e.g., Harris et al., 2014; Costoya et al., 2015) is an important climate change-related stressor impacting plankton communities. The increase in atmospheric carbon dioxide, which is primarily responsible for driving this warming, is also generating ocean acidification by increasing the flux of carbon dioxide across the ocean surface; however, there is still much uncertainty around the effects of ocean acidification on plankton communities (e.g., Beare et al., 2013; Beaugrand et al., 2013). Climate change is also altering local climate conditions such as wind speed (e.g., Rusu, 2022) and precipitation regimes (e.g. Rodríguez‐Puebla and Nieto 2010; Tsanis and Tapoglou, 2019), which also potentially impact light attenuation (Cappuzzo et al., 2015) and sea surface salinity (Holt et al., 2010).
Air and water temperatures in the Arctic waters of OSPAR Region I have been increasing since the late 1980s, with record high temperatures recorded in 2016 (IPCC; Lind et al., 2018). This prolonged warming trend is primarily due to a reduction in heat exchange from the ocean to the atmosphere (Skagseth et al., 2020). Boreal zooplankton (e.g., Calanus finmarchicus) has been increasing in abundance throughout eastern and northern areas of the Barents Sea (ICES WGIBAR, 2020). Future changes in temperature, ice cover, precipitation, freshwater run-off and wind are expected to affect both primary and secondary production as surface salinity and seasonal dynamics continue to change (von Quillfeldt et al., 2018). If summer stratification between deeper water and the surface layer continues to intensify, the surface layer may become increasingly nutrient-limited during the growing season, causing reductions in phytoplankton production and biomass and further reducing the energy available to support the rest of the food web (Li et al., 2004). Such changes, occurring at different spatial and temporal scales, will benefit smaller planktonic lifeforms such as flagellates and picocyanobacteria, which are of poor availability and lack the nutritional quality to support higher trophic levels (Schmidt et al., 2020). By contrast, any increase in mixing would provide more favourable conditions for supporting diatom production (Sakshaug, 2004). Stronger mixing can occur when strong winds and storms increase exchange between the surface layer and deeper water. This mixing replenishes nutrient concentrations in surface waters, facilitating productive conditions for microphytoplankton growth.
In the current assessment, sea surface warming has been statistically linked to changes in plankton communities, primarily within shelf and oceanic pelagic habitats, between 1960 and 2019. Indicator results for PH1/FW5 (Figure CC.1) reveal that increasing sea surface temperature is linked to declining abundances of planktonic lifeforms, particularly small and large copepods, across the North-East Atlantic. This trend was also reflected by the patterns in zooplankton abundance detected with the PH2 indicator over the same period. Decreasing trends in zooplankton abundance were probably also influenced by the decreasing trends in phytoplankton biomass and abundance; however, links between phytoplankton and zooplankton have not been explicitly tested in the current assessment.
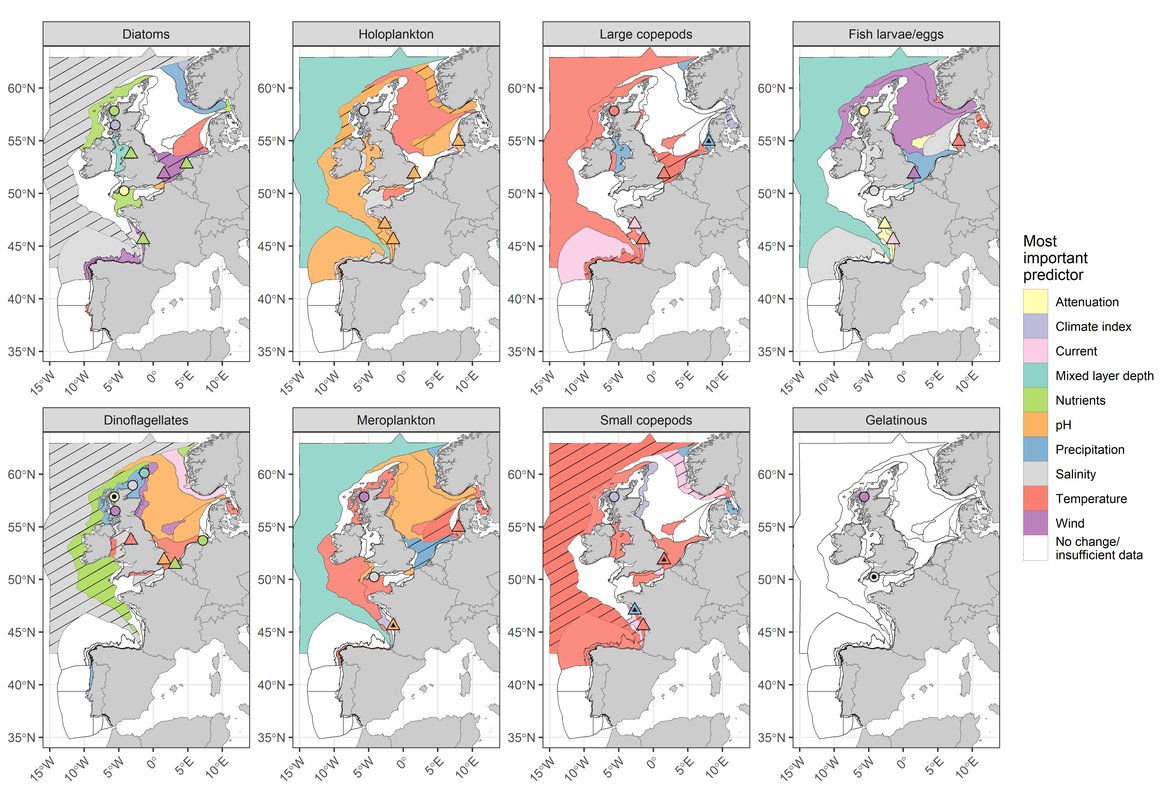
Figure CC1: PH1/FW5 indicator results displaying the most important environmental variable linked to abundance trends for eight planktonic lifeforms. For a detailed description of methods, please refer to the PH1/FW5 indicator assessment
Rising temperatures were also strongly linked to increasing meroplankton abundance and decreasing copepod abundance throughout the Greater North Sea between 1960 and 2019. The increase in meroplankton abundance, particularly that of the sea urchin Echinocardium cordatum, over holoplankton (primarily copepods) has been well studied since the early 2000s (Kirby et al., 2007; Kirby et al., 2008). The current assessment has identified changes in plankton community composition which are strongly linked with the increasing trend in sea surface temperature. The results from the pilot assessment of the PH3 candidate indicator in the Greater North Sea also support this conclusion. The increasing dominance of meroplankton identified by the PH1/FW5 indicator was also supported by the output from the pilot assessment of the PH3 candidate indicator, which detected a trend towards atypical zooplankton community composition in the current OSPAR assessment period (2015-2019) due to the high relative abundance of echinoderm larvae and post-larval stages.
At the scale of the four pelagic habitat types (i.e., variable salinity, coastal, shelf, oceanic / beyond shelf) the PH1/FW5 indicator has revealed that meroplankton increased in abundance primarily within coastal and shelf areas of the Greater North Sea, with the increasing trends closely linked to rising sea surface temperatures between 1960 and 2019. Simultaneous declines in the abundance of holoplankton in shelf regions have also been linked to the rise in temperature. In OSPAR Region III (Celtic Seas), declining abundances of dinoflagellates, and of large and small copepods in coastal areas, have been observed and are similarly linked to the rise in temperature. In shelf areas of this Region there has also been a strong increase in meroplankton abundance in response to temperature. Finally, zooplankton abundance (Figure CC.2B) and, more specifically, large and small copepod abundance (Figure CC.1), have declined in oceanic / beyond shelf habitats of Region IV (Bay of Biscay and Iberian Coast), concurrently with the rise in sea surface temperature.
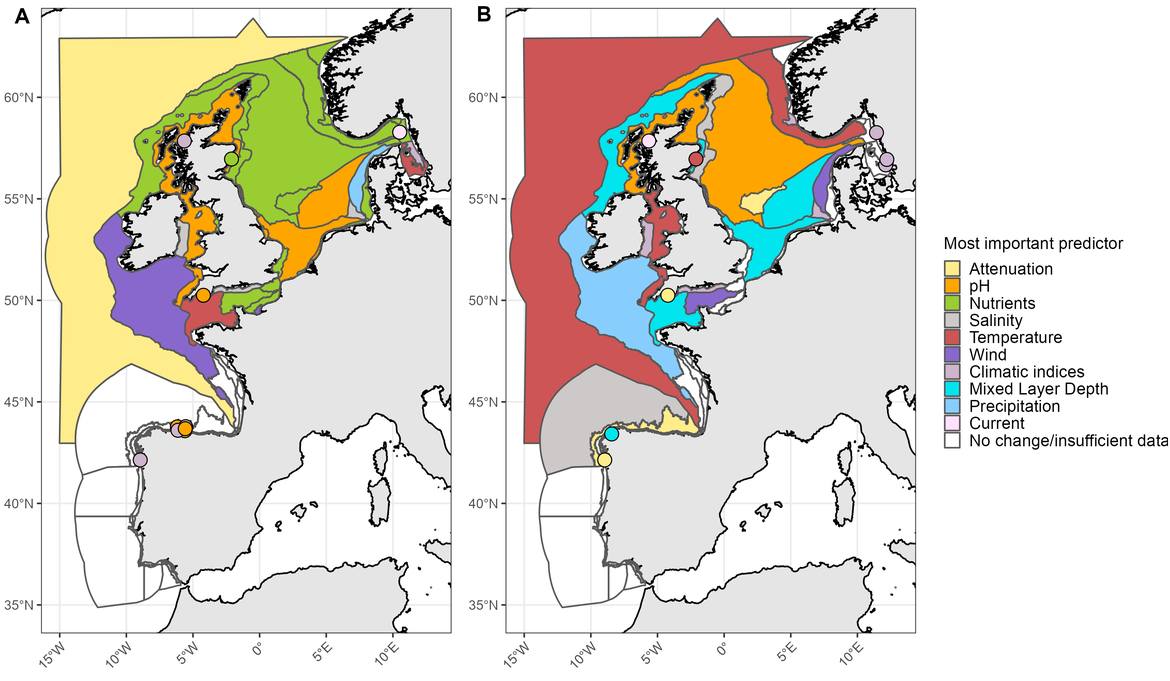
Figure CC2: PH2 indicator results displaying the most important environmental variable linked to trends in phytoplankton biomass (A) and zooplankton abundance (B). For a detailed description of methods, please refer to the PH2 indicator assessment
The pelagic habitats indicator assessments have collectively identified modelled pH as another factor which probably relates to the changes occurring in plankton communities, particularly within shelf and oceanic habitats. The pH level of seawater is controlled by the concentration of dissolved inorganic carbon (DIC). Changes in pH can be linked to changes in phytoplankton activity, since phytoplankton ingest DIC to fuel growth and reproduction, but also to the concentration of atmospheric carbon dioxide, the main driver of global warming. The Ocean Acidification Other Assessment found a pattern of widespread decline in pH across all OSPAR Regions. Open ocean areas are experiencing rates of pH decline between -0,001 to -0,002 yr-1, and up to -0,03 yr-1 along the near-shore coastline of the English Channel and Bay of Biscay. The rate of change also varies with depth, with the fastest decline occurring at the surface, due primarily to the influence of atmospheric carbon dioxide. At a more localised scale, trends in pH can show greater variation, and increasing pH trends still persist. Due to the high complexity of ocean acidification dynamics, it is important for future assessments to focus on untangling the primary drivers responsible for the net decline in pH, and to determine how their influence varies across OSPAR Regions and pelagic habitat types.
Indicator assessment results suggest that in the Celtic Seas, declining abundance of holoplankton in coastal and shelf areas between 1960 and 2019 may also be linked to effects of decreasing pH, while phytoplankton diversity is not affected (Figure CC.3A). The decrease in pH also co-occurred with a decline in phytoplankton biomass (Figure CC.2A) and zooplankton abundance (Figure CC.2B) within shelf habitats of the Celtic Seas. A reduction in phytoplankton biomass would also cause a reduction in the uptake of DIC from the water column (to be confirmed by the primary productivity food web indicator FW2), which could contribute to the observed decline in pH.
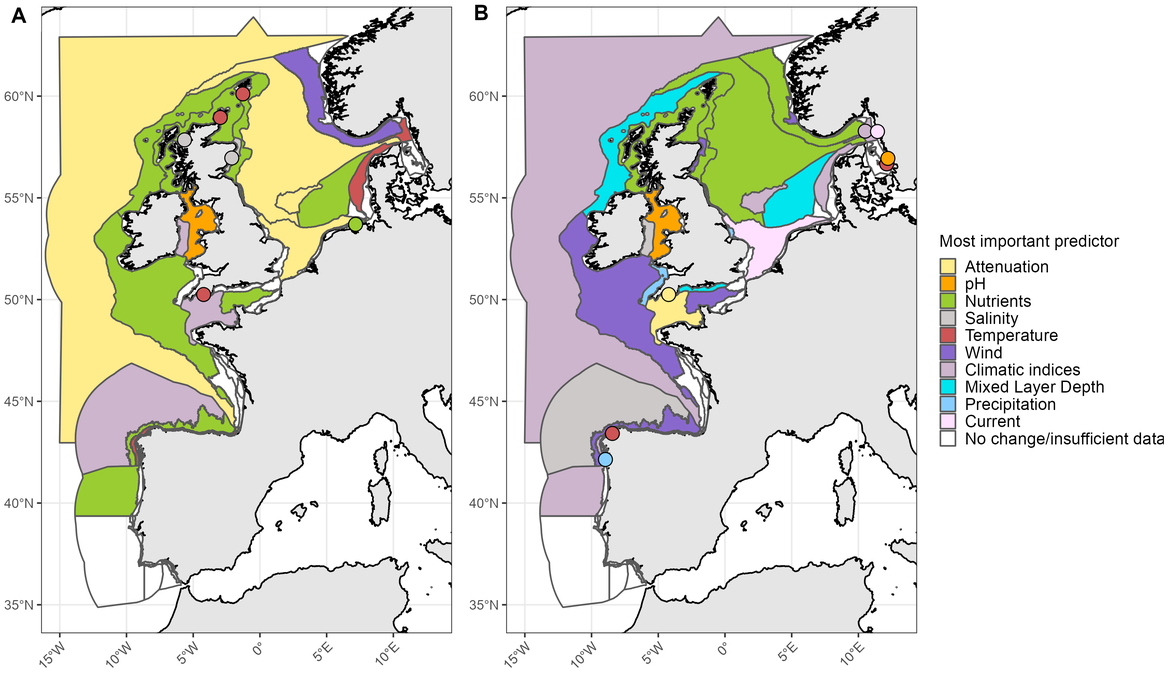
Figure CC.3: PH3 indicator results displaying the most important environmental variable linked to trends in phytoplankton diversity (A) and zooplankton diversity (B). For a detailed description of methods, please refer to the PH3 indicator assessment. Note that the PH3 is currently only considered a common indicator in the Celtic Seas (OSPAR Region III). However, a pilot assessment was also conducted for the Greater North Sea and the Bay of Biscay and Iberian Coast (OSPAR Regions II and IV, respectively). This is a hybrid figure showing results from the common indicator assessment for the Celtic Seas and for the pilot assessment for the Greater North Sea and the Bay of Biscay and Iberian Coast
In the Bay of Biscay and Iberian Coast Region, shelf areas exhibited declining holoplankton abundance from 1960 to 2019, also linked to the decrease in modelled pH. Across the OSPAR Maritime Area the holoplankton lifeform is primarily composed of copepods. While negative changes in pH can inhibit shell formation in pelagic gastropods and other calcareous zooplankton (Beare et al., 2013), these organisms do not contribute a large proportion of holoplankton abundance and are therefore unlikely to be responsible for driving net decreasing trends. It is likely that this apparent relationship between pH and holoplankton abundance is driven by the decline in phytoplankton biomass and the resulting decrease in the uptake of DIC by phytoplankton, or by the combined effects of other climatic, chemical, and biotic drivers (Beare et al., 2013).
In addition to driving ocean warming, climate change can lead to changes in sea surface salinity and light attenuation. However, climate change is not always responsible for driving changes in these variables. The PH3 indicator assessment suggested a decrease in zooplankton diversity associated with decreasing sea surface salinity in the Celtic Seas (Figure CC.3B). Similar observations were made for oceanic habitats of the Bay of Biscay and Iberian Coasts, where declining phytoplankton biomass in oceanic habitats was linked to changes in light attenuation (Figure CC.2A).
Changes in plankton communities (including lifeforms, biomass, abundance, and diversity) due to other anthropogenic influences (e.g., nutrient input from rivers) were less definitive, since there was high variability in the relative ranking of pressures according to their degree of influence on the pelagic habitat indicators.
Indicator assessment results reveal that climate change is probably the most important pressure currently impacting plankton communities, with the strongest effects detected in shelf and oceanic habitats. Considering the influence of climate change on nutrient availability in stratified waters and the co-occurring declines in phytoplankton detected throughout much of the OSPAR Maritime Area, it is increasingly important for future assessments to consider the links between trends in phytoplankton and zooplankton in the context of climate change. Global efforts to slow climate change will probably be the best mechanism to counter widespread changes in plankton communities occurring across these offshore pelagic habitats. Regionally targeted management measures, which include improved control over nutrient input from anthropogenic sources, are likely to generate noticeable impact only within variable salinity and coastal habitats, but may have some effect in areas where pelagic habitats are affected by the cumulative impacts of multiple pressures (i.e., warming and eutrophication).
Beare, D., McQuatters-Gollop, A., van der Hammen, T., Machiels, M., Teoh, S. J. and Hall-Spencer, J. M. (2013). Long-term trends in calcifying plankton and pH in the North Sea. PLoS One, 8(5), e61175.
Beaugrand, G., McQuatters-Gollop, A., Edwards, M. and Goberville, E. (2013). Long-term responses of North Atlantic calcifying plankton to climate change. Nature Climate Change, 3(3), 263-267.
Capuzzo, E., Stephens, D., Silva, T., Barry, J. and Forster, R. M. (2015). Decrease in water clarity of the southern and central North Sea during the 20th century. Global change biology, 21(6), 2206-2214.
Costoya, X., Decastro, M., Gómez-Gesteira, M. and Santos, F. (2015). Changes in sea surface temperature seasonality in the Bay of Biscay over the last decades (1982–2014). Journal of Marine Systems, 150, 91-101.
Hallegraeff, G. M., Anderson, D. M., Belin, C., Bottein, M. Y. D., Bresnan, E., Chinain, M. and Zingone, A. (2021). Perceived global increase in algal blooms is attributable to intensified monitoring and emerging bloom impacts. Communications Earth & Environment, 2(1), 1-10.
Harris, V., Edwards, M. and Olhede, S. C. (2014). Multidecadal Atlantic climate variability and its impact on marine pelagic communities. Journal of Marine Systems, 133, 55-69.
Holt, J., Wakelin, S., Lowe, J. and Tinker, J. (2010). The potential impacts of climate change on the hydrography of the northwest European continental shelf. Progress in Oceanography, 86(3-4), 361-379.
ICES 2020. Working Group on the Integrated Assessments of the Norwegian Sea (WGINOR; outputs from 2019 meeting). 52s. ICES Scientific Reports. 2:29. 46 s. http://doi.org/10.17895/ices.pub.5996
IPCC 2019: IPCC Special Report on the Ocean and Cryosphere in a Changing Climate [H-O. Pörtner, DC.
Kirby, R. R., Beaugrand, G. and Lindley, J. A. (2008). Climate‐induced effects on the meroplankton and the benthic‐pelagic ecology of the North Sea. Limnology and Oceanography, 53(5), 1805-1815.
Kirby, R. R., Beaugrand, G., Lindley, J. A., Richardson, A. J., Edwards, M. and Reid, P. C. (2007). Climate effects and benthic–pelagic coupling in the North Sea. Marine Ecology Progress Series, 330, 31-38.
Li, W.K.W., McLaughlin, F.A., Lovejoy, C. and Carmack, E.C. (2009). Smallest algae thrive as the Arctic Ocean freshens. Science 326, 539-539.
Lind, S., Ingvaldsen, R.B. and Furevik, T. (2018). Arctic warming hotspot in the northern Barents Sea linked to declining sea-ice import. Nature climate change, 8 (7), 634-639.
Rodríguez‐Puebla, C. and Nieto, S. (2010). Trends of precipitation over the Iberian Peninsula and the North Atlantic Oscillation under climate change conditions. International Journal of Climatology, 30(12), 1807-1815.
Rusu, E. (2022). Assessment of the wind power dynamics in the North Sea under climate change conditions. Renewable Energy, 195, 466-475.
Sakshaug E. (2004). Primary and secondary production in the Arctic Sea, in Stein, R. and Macdonald, R.W. (eds): The organic carbon cycle in the Arctic Ocean. Springer, Berlin, pp. 57-81.
Schmidt, K., Birchill, A. J., Atkinson, A., Brewin, R. J., Clark, J. R., Hickman, A. E. and Ussher, S. J. (2020). Increasing picocyanobacteria success in shelf waters contributes to long‐term food web degradation. Global Change Biology, 26(10), 5574-5587.
Skagseth, Ø., Eldevik, T., Årthun, M., Asbjørnsen, H., Lien, V. S. and Smedsrud, L. H. (2020). Reduced efficiency of the Barents Sea cooling machine. Nature Climate Change, 10(7), 661-666.
Tsanis, I. and Tapoglou, E. (2019). Winter North Atlantic Oscillation impact on European precipitation and drought under climate change. Theoretical and Applied Climatology, 135(1), 323-330.
von Quillfeldt, C. H. (2018). Miljøverdier og sårbarhet i iskantsonen.
Cumulative Effects | Executive Summary and Five Questions |