Marine climate change as a pressure
Anthropogenic greenhouse gas emissions are causing changes in the ocean climate. These can be seen as pressures on marine biodiversity and human activities. Across the OSPAR Maritime Area, these pressures are mounting: increased sea temperature, shrinking sea ice, increased freshwater input, changed salinity, changes in large-scale ocean circulation, shelf sea and open ocean stratification, increased storms and waves, rising sea level, reduced uptake of CO2, acidification, nutrient enrichment, reduction in dissolved oxygen, changes in upwelling intensity and marine heatwaves. Changes in these pressures have been identified in all OSPAR Regions, although Arctic Waters in particular are experiencing changes at much faster rates.
Note on confidence assessment: Due to the specific nature of the climate change Thematic Assessment, which is not backed by the assessment of agreed OSPAR indicators, adding an assessment of the confidence was considered irrelevant.
Human activities have caused major changes in the Earth’s climate system, with warming observed in the atmosphere, in the ocean and on land. The scale and rate of change is unprecedented in thousands of years. The ocean has absorbed 89% of the excess heat (von Schuckmann et al., 2020), and every year it absorbs at least a quarter of the carbon dioxide (CO2) released to the atmosphere (see: Ocean Acidification Other Assessment ). Due to human influence, the marine environment is warming, its dissolved oxygen concentration reducing and its pH content decreasing (acidification). This is also causing widespread change in the water cycle (changing prevailing atmospheric conditions) and causing changes in other parameters such as stratification. Climate extremes, including marine heatwaves and extreme weather events, are also becoming more prevalent. These changes in the physical and chemical conditions act as pressures on marine habitats and ecosystems, and on the human activities taking place across the OSPAR Maritime Area. Consequently, ecosystem service impacts due to climate change and ocean acidification will also occur. The following paragraphs provide further details of these pressures and how they have changed and may change in future, across the North-East Atlantic Ocean. They have also been summarised in the following infographic:
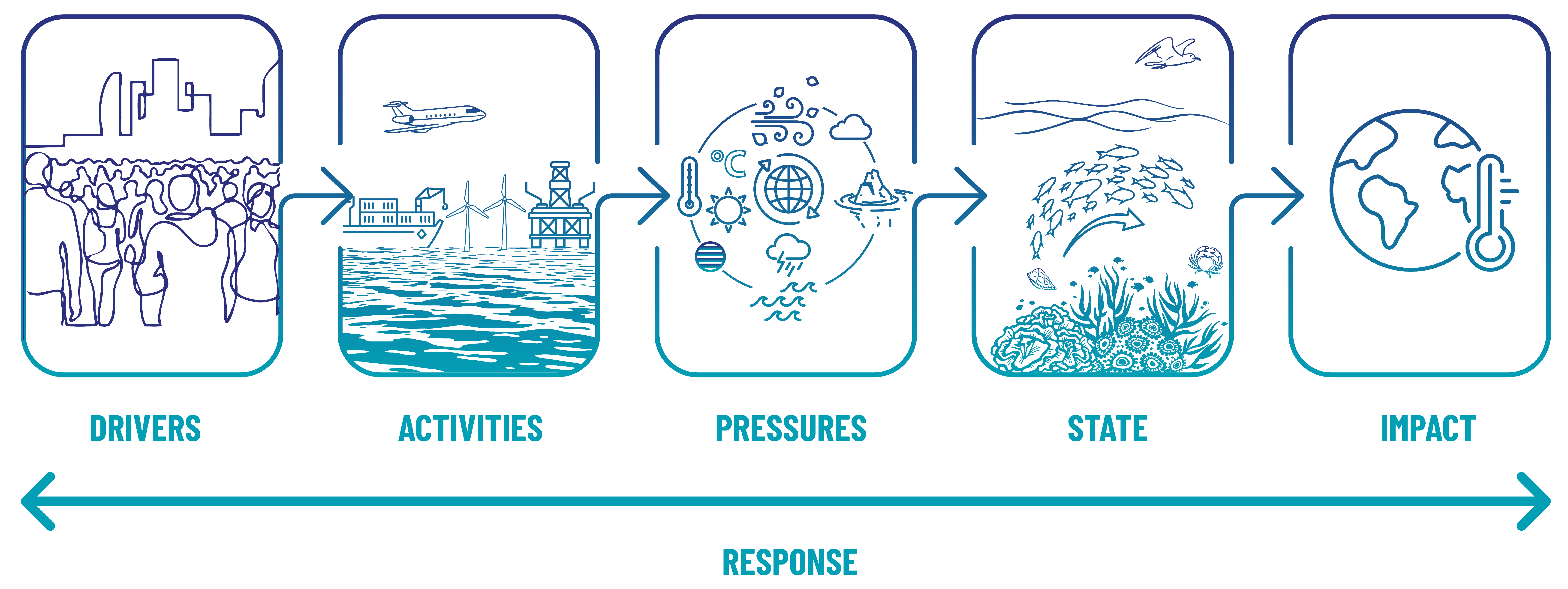
Increased sea temperatures
Increasing sea temperatures show the imprints of the global warming trend associated with anthropogenic climate change, as well as variability, on inter-annual to multi-decadal time scales, due to natural cycles, and differences between regions. Examination of the changes in temperature at regional scale in the North Atlantic, whether over the past century or the past 30 years, reveals that the temperature evolution at any particular location does not follow a smooth, continuously upward path (Figure P.1). Records show short periods of rapid warming, periods of little change, and periods of cooler conditions. Changes in sea surface temperature are presented below, followed by a more complex presentation of temperature change in the upper, intermediate and deep layers of the north-east Atlantic.
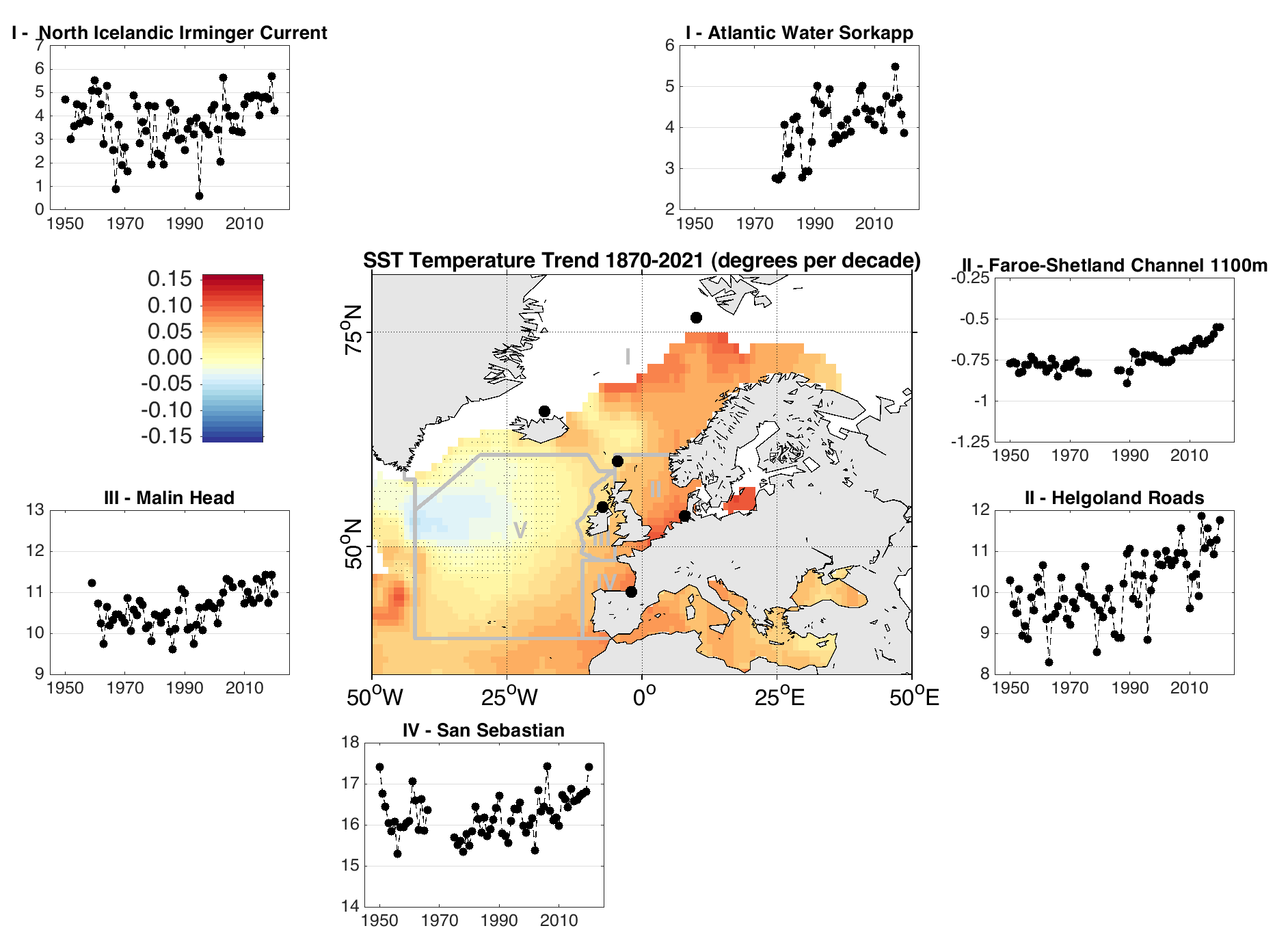
Figure P.1: Sea temperature trends at key locations in the OSPAR Maritime Area from HadSST sea surface temperature (Rayner et al., 2003), and selected time series from the ICES Report on Ocean Climate (Gonzalez-Pola et al., 2020)
Statistical significance of trends determined by Kendall rank correlation. Stippled areas show trend is not statistically significant. Data provided by Met Office – UK, Hafrannsoknastofnun - Marine Research Institute - Iceland, Institute of Marine Research - Norway, Marine Scotland Science – UK, Alfred-Wegener Institut Helmholtz-Zentrum für Polar- und Meeresforschung – Germany, Aquarium of San Sebastian (Oceanographic Society of Gipuzkoa and Oceanographic Foundation of Gipuzkoa) – Spain, Marine Institute/Met Eireann - Ireland)
The recent Intergovernmental Panel on Climate Change (IPCC) assessment report has stated: “it is certain that global sea surface temperature (SST) has increased since the beginning of the 20th century” (Fox-Kemper et al., 2021). Estimates from the 5th issue of the Ocean State Report (von Schuckmann et al., 2021) suggest that the global ocean has warmed by 0,015 ± 0,001 °C/year between 1993 and 2019. The north-west shelf region of Europe broadly mirrors the global trend of warming in sea surface temperature (0,017 ± 0,001 °C/year).
The International Council for the Exploration of the Sea (ICES) Working Group on Oceanic Hydrography provides more regional context for changes in ocean temperature based on long-established ocean sections, some of which are a century or more in duration (Figure P.1). In the Greenland Sea, the upper 100 m of the ocean warmed over the 2013 to 2018 period, and relative cooling has been observed since 2018. By contrast, the Norwegian basin experienced a strong influence of warm and saline Atlantic waters between 2006 and 2016, with the Atlantic waters becoming fresher since 2016 (Skagseth et al., 2022). That predominance of Atlantic waters is consistent with “Atlantification” and associated reduced sea-ice cover in the Atlantic sector of the Arctic Ocean (Barents Sea, Fram Strait) (Asbjørnsen et al., 2020; von Schuckmann et al., 2021). Temperature time series derived from ocean quahog clam shells for coastal regions of Norway in the Barents Sea imply a warming of at least 2 °C from the mid-18th century to 2014 (Mette et al., 2021). The Irminger Basin (south-west of Iceland) has exhibited warming since 2018, reversing the previous trend associated with the subpolar North Atlantic cold anomaly between 2013 and 2016 (Josey et al., 2018).
The eastern Atlantic region has shown a decrease in upper ocean temperatures in recent years (ICES Working Group on Oceanic Hydrography). Those cooler surface temperatures were also recorded in the western Iberian margin upwelling region (OSPAR Region IV) and in the Canary upwelling region, although the long-term trend between 1982 and 2019 indicates warming in both regions with a rate of +0,0105 °C/year in OSPAR Region IV (Siemer et al., 2021). The underlying long-term warming trend is already leading to tropicalization of shallow marine communities in the southern areas of OSPAR Regions IV and V (e.g. Vergés et al., 2014; Schäfer et al., 2019). Temperatures around the Azores (Region V) have increased since 1982, reaching a maximum rate of 0,027 °C/year (Siemer et al., 2021). This trend was also recorded at subsurface level, with waters in the upper 240 m warming since 1998 and those at 500 m depth since 2006 (Fründt et al., 2013). Warmer (and more saline) central waters (200 to 400m) have also been observed in OSPAR Region IV since 2008 (Valente et al., 2019).
Contrary to the ascertained global ocean trend of warming down to 2000 m water depth since 1971 (Bindoff et al., 2019) and a likely warming trend below 2000 m water depth since 1992 (Fox-Kemper et al., 2021), the Irminger Sea has seen cooling of around 0,3° C in the 700 to 1500 m depth range. That cooling between 2016 and 2021 interrupted the warming phase that had prevailed since 1990 (Desbruyères et al., 2022) and might be related to the North Atlantic cold anomaly. The Greenland Sea has seen a positive warming trend since 2002.
Slight cooling has been apparent in the sub-tropical gyre (OSPAR Region V) since 2012 at depths between 700 and 1500 m (Gonzalez-Pola et al., 2020) and could be related to the long-term cooling trend observed in the Mediterranean Outflow Water since 1981 (Frazão and Waniek, 2021). Comparison of data from the 1980s to the 2000s shows, however, significant moderate warming at depths of 1000 and 1600 m in the south-eastern sector of OSPAR Region V, but no change at 3000 m (Frazão et al., 2021).
Reducing sea ice
Global warming is causing a reduction in sea ice in OSPAR Region I. The Arctic sea ice extent, the area covered at a particular time of year, has continued to show a decreasing trend (a 43% decline between 1979 and 2019; AMAP, 2021a). These declines have been significant in all months, although the largest reductions have been seen in September when the Arctic sea ice is at its lowest (Eyring et al., 2021). In the seasonal sea ice zone, the earlier onset of surface melt in spring and delay in the autumn freeze-up are lengthening the open water season, although exact quantification of regional trends is difficult owing to differences between observational products) (Fox-Kemper et al., 2021).
At least half of the change in the Arctic sea ice can be attributed directly to human greenhouse gas emissions, with other causes including increased surface heat flux and increased Atlantic water influence (Hwang et al., 2020). Sea-ice thickness has been more difficult to monitor, but observations show that in the central Arctic Ocean it reduced by 65% over the period 1975 to 2012 (AMAP, 2017). Sea ice cover has also been thinning and reducing in age since the 1980s (AMAP, 2021a). Changes in the polar sea ice extent will create enhanced warming in the region (since water absorbs more sunlight than ice) and cause changes to the ecosystem, from primary producers to species composition, spatial distribution and abundance of species across the marine food web (Meredith et al., 2019).
Changes in the extent of the Arctic sea ice may also impact conditions beyond the Arctic Circle, including north-west Europe (Screen, 2021). The precise extent of and processes behind these changes remain active areas of research, with observational evidence and computer models showing low consensus (Cohen et al., 2020). One potential effect of sea-ice loss could be weakening and greater instability of the polar vortex and jet stream (two important strong winds in the atmosphere), which may cause extreme regional weather in the OSPAR Contracting Parties.
Elsewhere in the OSPAR Maritime Area, sea ice has been known to form in shallow parts of the southern North Sea under specific atmospheric conditions in wintertime. There has been a general decreasing trend in occurrences of North Sea ice winters since the mid-20th century, although conditions in the winters of 2010, 2011, 2012 resulted in some ice cover following a decade in which it had been absent (Brander et al., 2016; BSH, 2021).
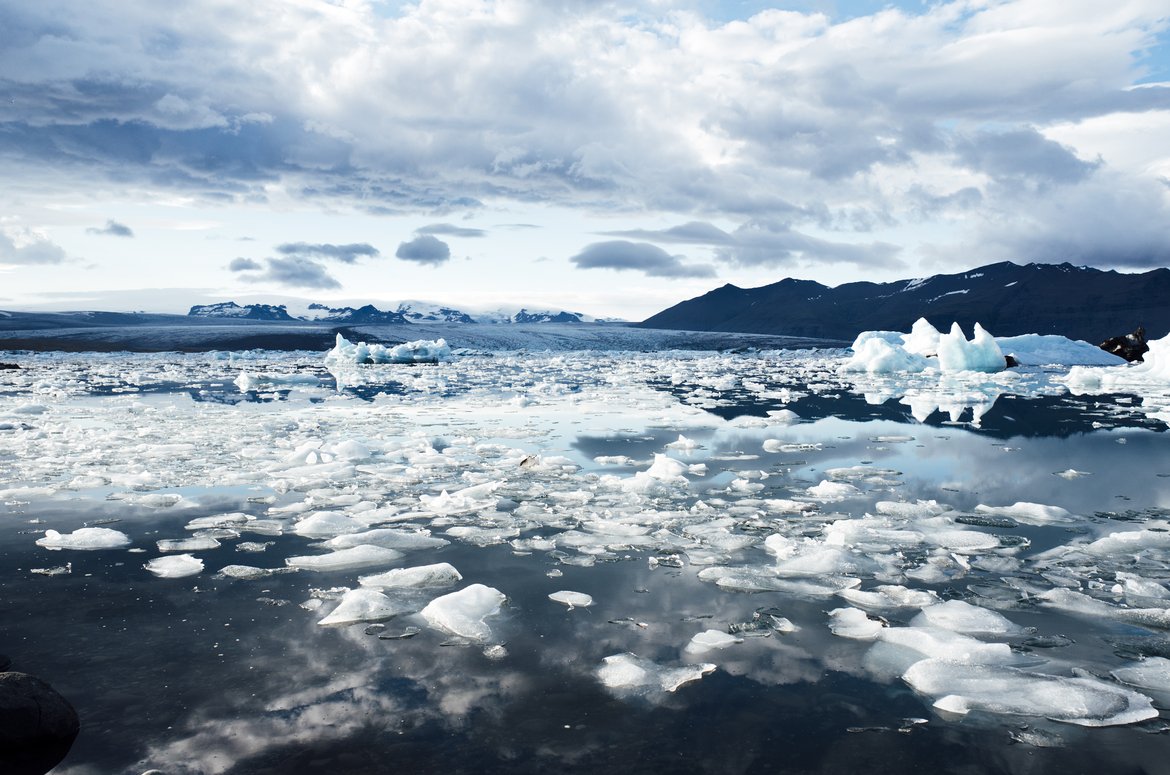
Global warming is causing a reduction in sea ice. © Pexels
Increased freshwater input
Global warming causes changes to the water cycle: in a warmer climate, the amount and intensity of rainfall events will increase and therefore freshwater inputs from precipitation and river run-off will increase. The melting of land-based ice (e.g. Greenland ice sheet and glaciers) will also increase freshwater input to the marine environment.
Observed precipitation trends across Europe since 1979 are not consistent across datasets, but analysis of longer records shows significant increases in precipitation for much of Scandinavia and north-western Europe (Fox-Kemper et al., 2021). Model-based estimates of river flow (European Environment Agency, 2016) show increased annual flows from land areas adjacent to Regions I (Arctic Waters), II (Greater North Sea) and III (Celtic Sea), but decreased river flow from those adjacent to Region IV (Bay of Biscay & Iberian Coast). Increased annual river flows are mainly associated with wetter winters, while decreasing trends in annual river flow are associated with drier spring and summer seasons. Under future climate change scenarios, these trends are expected to continue, with generally lower annual mean river flow in Region IV and higher annual mean river flow in Regions I, II and III. River discharges from the surrounding land increased in Region I between 1976 and 2017 (Meredith et al., 2019), and these trends are projected to continue.
Extreme rainfall events, such as those seen in parts of Germany, Belgium and the Netherlands in July 2021, have been attributed to climate change. As global warming continues, both the intensity and likelihood of occurrence of these rainfall extremes will continue to change. Although catchment dynamics, urbanisation, water management and other factors will influence how these rainfall events translate to river flow changes, it is probable that the likelihood and intensity of freshwater pulses in the marine environment will increase, particularly for Regions II and III, but also the coastal regions of Region I.
The contribution of freshwater from the melting of land-based ice will particularly influence Region I of the OSPAR Maritime Area. Reductions in land ice have been observed across Region I, with an increase in the rate of loss in recent decades (AMAP, 2021a). This loss of land ice in Region I is the largest regional contribution to global sea-level rise (AMAP, 2021b).
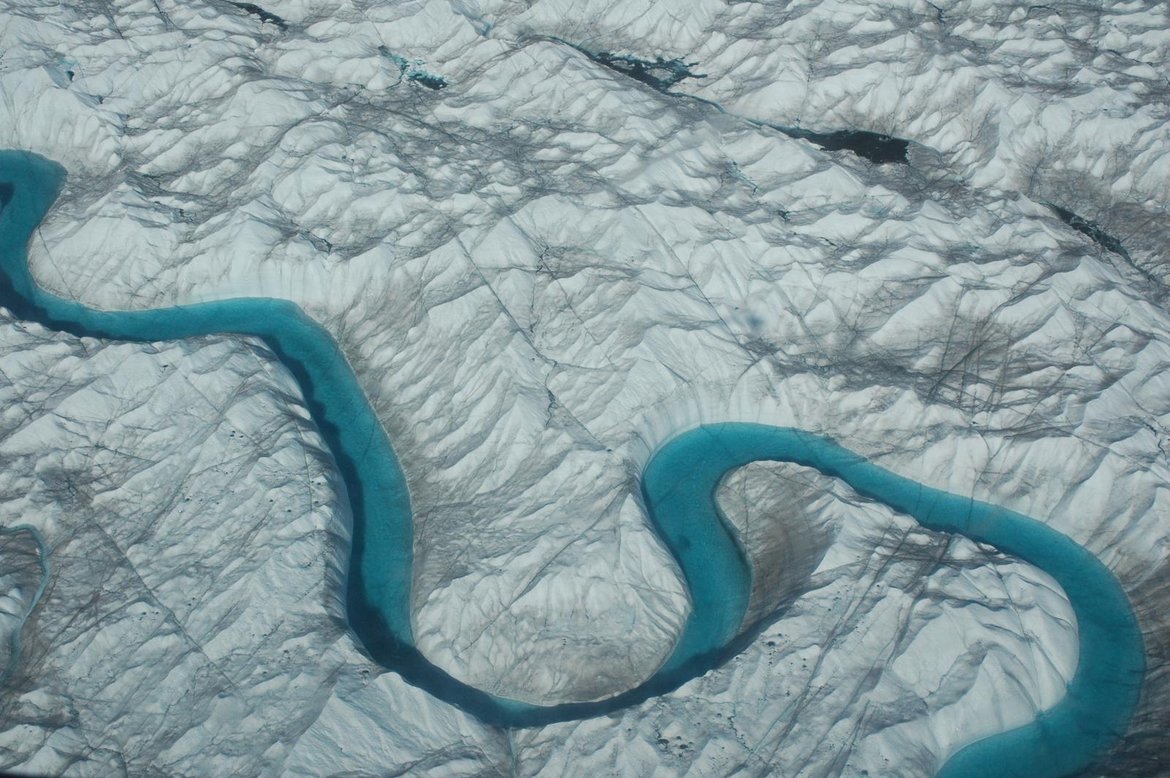
The melting of land-based ice like that seen here in Greenland will also increase freshwater input to the marine environment
Changed salinity
On a global scale, regions of higher salinity and evaporation (the tropics and subtropics) have become saltier, whereas regions of low salinity and greater precipitation have trended fresher since the 1950s (Durack, 2015; Bindoff et al., 2019). Those trends will continue into the 21st century (medium confidence) and reflect the increased intensity of the Earth’s hydrologic cycle (Durack, 2015; Eyring et al., 2021).
Freshening is observed in and forecast for the Arctic (Region I) due to increased freshwater flux from land (Bindoff et al., 2019; Fox-Kemper et al., 2021). There is high confidence that, at annual to decadal time scales, regional salinity changes are being driven by ocean circulation change superimposed on longer-term trends. Due to prevailing ocean currents, the salinities in the OSPAR Maritime Area have been strongly influenced by oceanic variability in the wider North Atlantic basin. For example, between the early 1990s and early 2010s, northward transport of warmer and saltier Atlantic waters from OSPAR Region V (Wider Atlantic) into the subpolar gyre (NW sector of Region V) and into Region I (Daniault et al., 2016; Lozier et al., 2019; Li et al., 2021) compensated for the salinity decrease expected from increased Greenland freshwater run-off in the subpolar North Atlantic (Fox-Kemper et al. 2021; Gonzalez-Pola et al. 2020). Salinities across the eastern subpolar North Atlantic Ocean have become fresher since 2015 (Holliday et al., 2020; Gonzalez-Pola et al., 2020), and this pattern is reflected in the observational records across the OSPAR Maritime Area (e.g. Norwegian Sea, Skagseth et al., 2022).
At depth, there is less of a consistent pattern across the OSPAR Maritime Area, again due to prevailing oceanographic circulation. The deep outflow from the Norwegian Sea recorded at the Faroe Bank Channel became saltier (and warmer) during the period 1995-2015 (Hansen et al., 2016). On the other hand, the deep water formed in 2015 and 2016 in the Irminger Sea (NW sector of Region V) resulted in lower salinities at depths between 1500 m and 2000 m in the Irminger Sea and the Labrador Sea (Lozier et al., 2019), in line with observed cooling. In the south-eastern sector of Region V, the intermediate depth (700 to 1500 m) Mediterranean Outflow Water not only became cooler, but also fresher, in the 1981 to 2018 period (Frazão and Waniek, 2021).
Changes in large-scale ocean circulation (incl. overturning)
The ocean’s circulation and its transport of heat, salt and other properties are critical components of the Earth’s climate system. Within the OSPAR Maritime Area, the Atlantic Ocean’s surface and deep ocean currents and their exchanges with the Arctic Mediterranean (i.e. the Arctic Ocean, Nordic Seas and adjacent shelf seas) play an important role in the climate of north-western Europe, as well as the productivity of the marine ecosystem. This is also called the Atlantic Meridional Overturning Circulation (AMOC). Based on palaeoceanographic reconstructions, the AMOC appears to have remained relatively stable during the past 8 000 years (IPCC AR6 WG1, 2021; medium confidence). The AMOC appears to be weaker than in the pre-industrial era, although there is no evidence to identify the magnitude of this weakening or to attribute it to anthropogenic greenhouse gas emissions (Garcia-Soto et al., 2021). More recently, historical ship-based hydrographic estimates of the AMOC (1980s onwards) show no overall decline in AMOC strength (Fu et al., 2020; Worthington et al., 2021). Since the mid-2000s, direct observational evidence of AMOC strength in the sub-tropical North Atlantic Ocean (RAPID-MOCHA-26N) has increased our understanding of the AMOC’s variability over shorter time scales (seasonal to decadal). These direct observations have identified a reduction in AMOC strength over decadal time scales (e.g. Mercier et al., 2015; Smeed et al., 2018). The directly observed weakening in the AMOC cannot be attributed to a long-term trend (high confidence; Moat et al., 2020). Based on the latest evidence, it is very likely that the AMOC will decline during the 21st century, but there is low confidence in the expected timing and magnitude. This weakening could be further enhanced by increased melting of the Greenland Ice Sheet (Fox-Kemper et al., 2021). The collapse of the AMOC is regarded as a climate tipping point (Collins et al., 2019), although considered very unlikely but plausible.
Since 2014, new direct measurements of the overturning circulation in the subpolar North Atlantic (part of the Overturning in the Subpolar North Atlantic Program; OSNAP) have improved our understanding of the overturning circulation in the subpolar North Atlantic (OSPAR Region V). Variability in the AMOC is strongly influenced by the conversion of surface to deep waters in the region between Greenland and Scotland (Lozier et al., 2019, Li et al., 2021). The large-scale circulation of the North Atlantic subpolar gyre (both in terms of the overturning and the horizontal gyre circulation) is important in setting the prevailing ocean climate across much of the OSPAR Maritime Area. Past changes in salinity and temperature across the region (see above) have been linked to changes in the strength of the subpolar gyre (for example, Häkkinen et al., 2011). Between the mid-2000s and mid-2010s, the subpolar gyre appeared to be in a weaker state, but in the past decade the circulation has been stronger (Berx, in prep; Gonzalez-Pola et al., 2020).
Changes in shelf sea stratification
Seasonally, the imbalance between mixing due to natural processes (such as tides and winds) and buoyancy changes from surface heating or freshwater results in some areas of shelf seas becoming stratified (a layer of more buoyant water overlies denser waters) and others becoming fully mixed. This process is most relevant in the shallow shelf regions of OSPAR Regions II and III. These areas of stratification have an impact on circulation and on many components of the ecosystem, for example by limiting the dispersal of larvae or by reducing nutrient supply between the near-surface and deeper layers. Seasonal stratification, both in terms of strength and duration, has been identified as important for our understanding of changes in the dissolved oxygen concentrations of seawater close to the seabed. In stratified areas, oxygen exchange from the atmosphere to this deep layer may be limited, leading to reduced dissolved oxygen concentrations (Sharples et al., 2020). There is no further update available on observed changes to strength and duration in shelf sea stratification. There is currently also no direct monitoring programme of shelf sea stratification taking place in the OSPAR Maritime Area. Models of future stratification in Regions II and III consistently project an increase in both the strength of stratification and in its seasonal duration as the regional climate changes through the 21st century (Lowe et al., 2009; Holt et al., 2010; Sharples et al., 2013).
Changes in open ocean stratification
Although studies focused on observed changes in open ocean stratification have been lacking until very recently, new works have increased our confidence in the rate of stratification change (Somavilla et al., 2017; Yamaguchi and Suga, 2019; Li et al., 2020; Sallée et al., 2021). The estimated density contrast across the mixed layer base, one metric of stratification, has been increasing during the past 60 years at varying global mean rates of 1 to 9% per decade (Yamaguchi and Suga, 2019; Li et al., 2020a; Sallée et al., 2021), while the mixed layer has deepened at a rate of several metres per decade (Somavilla et al., 2017; Sallée et al., 2021). Similar patterns of increasing stratification together with deepening of mixed layer depths have been observed in the open ocean across Region V and the wider North Atlantic. However, in the North Atlantic, stratification is increasing at slower rates (approximately half of the global average). This is due to the global signal being dominated by contributions from tropical regions (Yamaguchi and Suga, 2019; Li et al., 2020; Sallée et al., 2021), while the mixed-layer depth is deepening at trends similar to the global ocean at rates between 5 and 8 m per decade (Somavilla et al., 2017; Sallée et al., 2021).
Increased storms and waves
The observational evidence of the trends in atmospheric patterns and storminess reveals mixed results. There is low confidence in long-term (centennial) changes in storminess, although analysis of the observational evidence since the mid-1970s has identified increases in the frequency and intensity of the strongest storms in the North Atlantic (Wolf et al., 2020). Models and observations show an associated increase in annual and winter mean significant wave heights in the North-East Atlantic since the 1950s. Climate models show a low level of agreement on the future response of the North Atlantic storm track, although a continued poleward shift is likely. This is mainly due to the challenges of accurately representing the winter storm track into Europe, due to model biases and large natural variability. Under a high-emissions scenario, there could be an overall reduction in mean significant wave height in the North Atlantic by 2100, although the most severe waves could increase in height (Wolf et al., 2020).
In Region I, increases in high latitude storminess have been detected (AMAP, 2021b), the changes being associated with increased storminess in late autumn and winter due to a northward shift of the prevailing storm track. Owing to the difficulty in resolving and documenting polar lows, there is little evidence of a significant change in their occurrence. Future projections of climate change do not show a consistent signal of increases in storminess across Region I (AMAP, 2021b). However, given the suggested northward shift of the storm tracks, and the projected increases in heat and moisture, increased storm activity in Region I is plausible (AMAP, 2021b). The increase in the ice-free area may affect storms and weather at lower latitudes, due to increased wind and wave fetch and moisture exchange to the atmosphere (AMAP, 2021b).
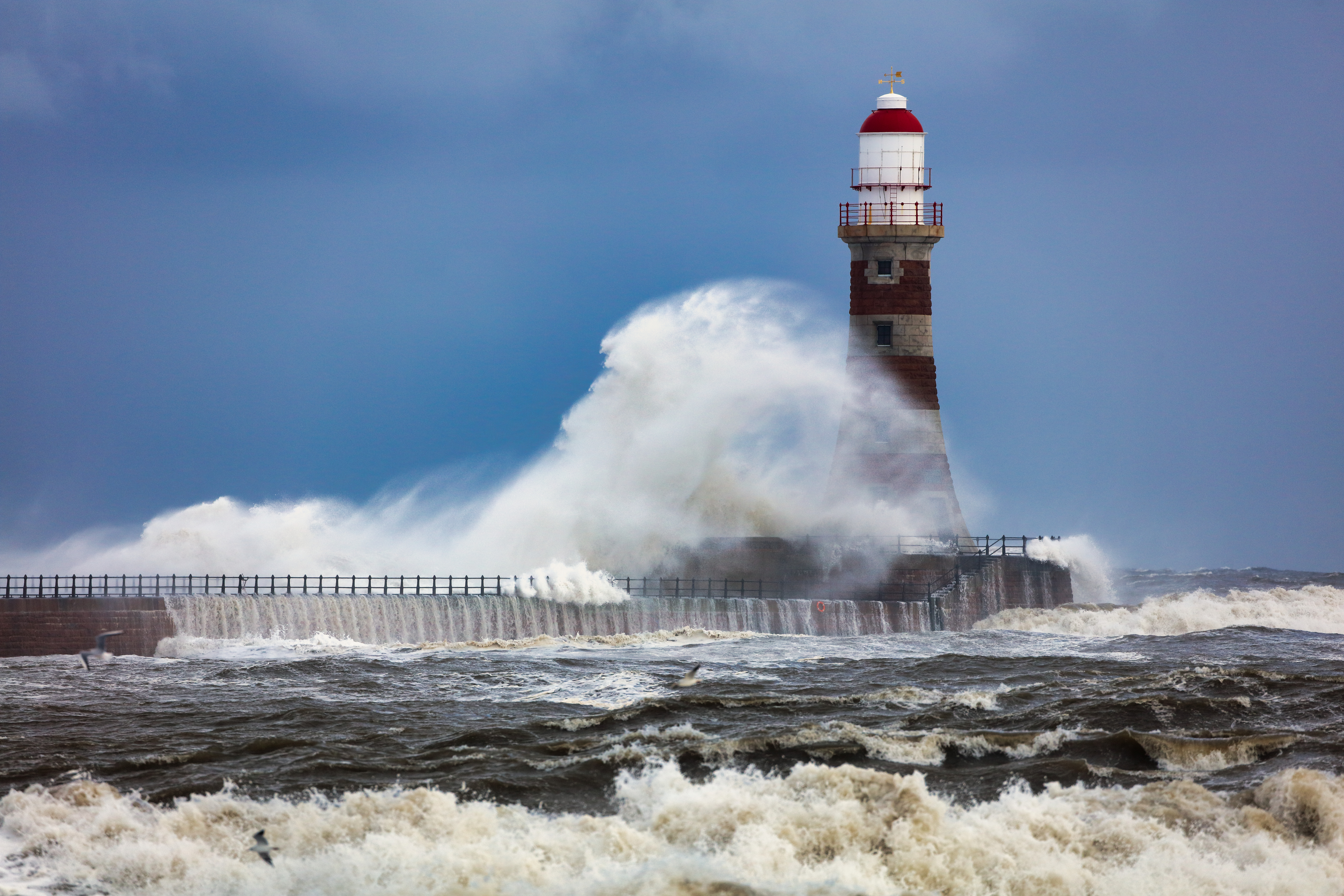
Analysis of the observational evidence since the mid-1970s has identified increases in the frequency and intensity of the strongest storms in the North Atlantic. © Shutterstock
Rising sea level
Global mean sea level rose by 0,2 m between 1901 and 2018. Between 1901 and 1971 the rate of sea level rise was 1,3 mm/year, and a higher rate of 3,7 mm/year has been observed by satellites in the recent two decades (2006 to 2018) (IPCC, 2021). The rise in sea level since 1900 has been faster than in any century over the last 3 000 years. This is reflected across European coastlines, albeit generally to a lower extent in northern areas that are experiencing a higher rate of post-glacial land rebound. Sea-level rise is estimated to be 3,6 ± 0,82 mm/year along the coasts of the Iberian peninsula, the Bay of Biscay and Ireland (Region IV and part of Region III) and 2,9 ± 0,83 mm/year between 1993 and 2020 for Europe’s north-west shelf (Regions II and III; von Schuckmann et al., 2021).
The impact of rising sea level is most evident when the local sea level combines with tidal, wave and weather conditions in an extreme high water level event. Increased occurrence of extreme high water level events in many locations is due to rise in local sea levels, rather than any change in storm or surge activity (EEA, 2014). North-west Europe in particular is projected to be a “hotspot” for significant changes in episodic flooding by 2100 (Kirezci et al., 2020).
In the period 2006 to 2015, meltwater from land-based ice in the Arctic (glaciers and ice caps) was the largest regional source of global sea-level rise (i.e. when not considering thermal expansion globally) (AMAP, 2021b; Oppenheimer et al., 2019). The rate of loss in the last two decades has been almost twice that prior to the mid-2000s (Meredith et al., 2019).
Global mean sea-level rise is projected to range from 0,37 to 0,86 m (5th-95th percentile range) by 2100 under the highest future CO2 emission scenario (IPCC, AR6). Due to factors such as vertical land motion, ocean circulation and water density changes, different regional patterns in local sea-level rise will occur around the OSPAR Maritime Area’s coastline. Vousdoukas et al. (2017) reported average projected increases of 0,57 to0, 81 m in a 100-year extreme sea-level event for Europe under varying emission pathways, including the OSPAR Maritime Area from Gibraltar to the Barents Sea coast of Norway. The North Sea region could face the highest increase in extreme sea levels (approximately 1 m under a high emissions climate scenario by 2100), followed by the Baltic Sea and the Atlantic coasts of the United Kingdom and Ireland. The projected sea-level rise within the IPCC’s sixth assessment cycle is higher than that it had reported previously, and therefore higher than the estimates reported in QSR 2010 and IA 2017. This is due to an improved understanding and representation of the key processes.
Reduced uptake of CO2
The ocean mitigates climate change by taking up anthropogenic CO2. A high-quality assessment of the ocean carbon sink is critical for assessing changes in the contemporary carbon cycle and for a robust projection of its future evolution. Sudden changes in the ocean carbon sink would immediately affect the allowable emissions for limiting global warming to well below 2 °C (Hauck et al., 2020). For the Nordic Seas, Olafsson et al. (2009) and Skjelvan et al. (2008; 2021) have shown that the sea surface pCO2 undersaturation is weakening and thus that the CO2 uptake from the atmosphere is reduced.
The ocean CO2 sink has been estimated at 2,8 ± 0,4 GtC yr-1 for the decade 2011 to 2020 (26% of total CO2 emissions), and the preliminary estimate for 2021 is approximately 2,9 GtC yr-1 (Friedlingstein et al., 2021). Observational data from Ships of Opportunity shows that the mid-latitude North Atlantic Ocean around the Porcupine Abyssal Plain (a long-term monitoring site in Region V) has remained a sink for atmospheric CO2 in recent years. (Macovei et.al., 2020).
Ocean circulation variability has been the primary driver of these changes in oceanic CO2 uptake over the past several decades. Multiple factors influence ocean CO2 uptake rates, including sea surface temperature (SST) and chemistry, biological CO2 utilization, and ocean circulation patterns. Measured sea surface CO2 concentrations integrate all these factors, making it difficult to disentangle the influence of each on the changing oceanic CO2 sink (De Vries et al., 2017).
Acidification
The ocean’s uptake of approximately one quarter of the CO2 released to the atmosphere by human activities each year results in a chemical reaction that reduces pH and aragonite saturation state, also called ocean acidification . The rate of acidification is highly local, with differences both between and within regions.
Open water pH has decreased by -0,001 to -0,002 yr -1, but coastal time series in the English Channel (Region II) and Bay of Biscay (Region IV) show declining pH rates of -0,03 yr -1. Short-term variability is large, especially in coastal regions, due to both natural and anthropogenic processes. These may mask the long-term signal owing to anthropogenic CO2 emissions. Sustained time series remain limited, and OSPAR will implement an ocean acidification monitoring strategy as part of NEAES 2030 (S10.O1).
The most effective way to limit future ocean acidification is to reduce CO2 emissions. Future climate projections estimate the average trend of ocean acidification to be -0,0017 yr -1 in Region I, and between -0,0021 and -0,0023 yr -1 elsewhere in the OSPAR Maritime Area, under a medium emissions scenario (see: Ocean Acidification Other Assessment ). These climate projections also estimate that a small part of the shallow shelf will be corrosive for unprotected calcareous organisms. Under a high emissions scenario, these acidification rates will be higher, and the corrosive waters will expand to a large part of the seafloor.
A more detailed assessment of acidification in the OSPAR Maritime Area, current state and future trends, can be found in the Ocean Acidification Other Assessment .
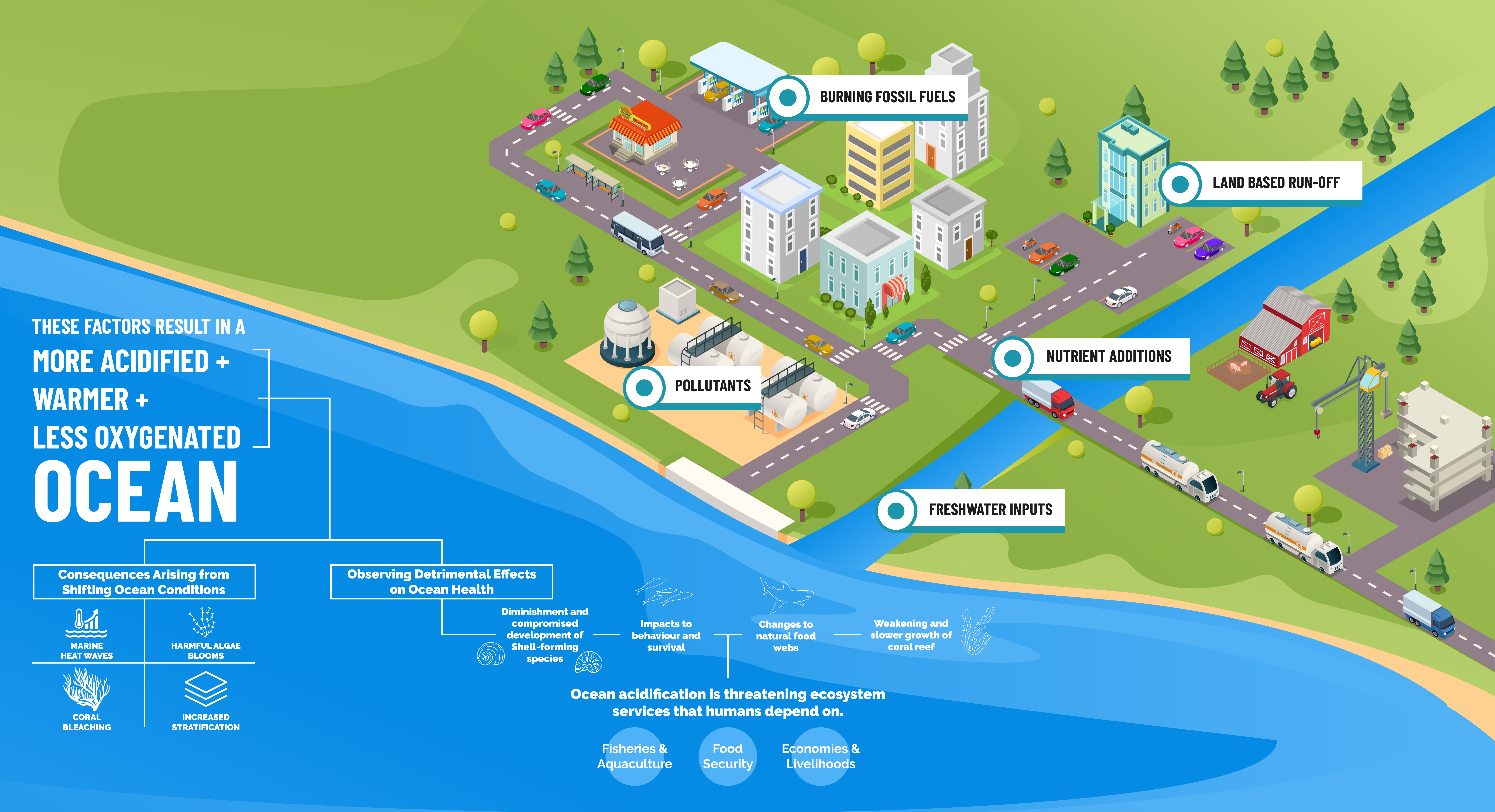
Nutrient enrichment
Good status was achieved in the majority of the OSPAR Maritime Area (see: Eutrophication Thematic Assessment ), although the overall ‘not good’ status assessment for river plumes in Regions II and IV demonstrates the continued need to reduce eutrophication effects. With respect to management, reductions in point source inputs from sewage treatment plants are proving increasingly successful. However, controlling the inputs from diffuse sources remains a challenging problem. This requires sustained, integrated research and monitoring, as well as repeated assessments of nutrient loading and impacts (Malone & Newton, 2020). Global climate changes will likely result in higher water temperatures and stronger stratification, and increased inflows of freshwater and nutrients to coastal waters may increase the inputs of nutrients to the marine and coastal environment, with consequences for eutrophication and marine biota. These resulting changes are not straightforward to predict, in part due to uncertainties about future land use and socio-economic developments.
Reducing dissolved oxygen
Climate change has an impact on the amount of oxygen that can be dissolved in seawater: ocean warming reduces the amount of oxygen that can be held, and ocean stratification limits the oxygen concentration in deeper layers. Increased levels of carbon dioxide can also promote phytoplankton growth, which, when it decays and is consumed beneath the thermocline, can further decrease oxygen concentrations (see: Dissolved Oxygen near the Seafloor Indicator Assessment ).
There is a growing consensus that between 1970 and 2010 the open ocean very likely lost 0,5 to 3,3% of its dissolved oxygen in the upper 1 000 m, and that oxygen minimum zones in the global ocean are expanding (Bindoff et al., 2019; Canadell et al., 2021). In coastal ocean regions, anthropogenic eutrophication via continental run-off and atmospheric nutrient deposition, as well as ocean warming, are very likely the main drivers of deoxygenation (Bindoff et al., 2019; Canadell et al., 2021).
In European shelf seas, most areas (except for the Baltic Sea) become vertically well mixed in winter every year. This resets oxygen concentrations to the surface values. As oxygen consumption is mainly driven from close to the seabed, the regions which suffer from low oxygen concentrations are those which have a relatively small volume of water beneath the thermocline. If climate change extends the length of time that regions remain isolated from the atmosphere (i.e. a longer time between the onset of stratification and the breakdown of stratification), this will also increase oxygen utilisation.
There is no observed widespread oxygen deficiency across most of the OSPAR Maritime Area, although more localised areas of oxygen deficiency are present in Regions II and IV (see: Dissolved Oxygen near the Seafloor Indicator Assessment ). There are statistically significant trends of reducing oxygen concentration in five regions (Atlantic, Atlantic Seasonally Stratified, Meuse, Scheldt Plume, Norwegian Trench) and of increasing oxygen concentration in one (Kattegat Coastal). Among these, it is only the Atlantic Seasonally Stratified region where reduced solubility due to increased sea temperature is the dominant effect (see: Dissolved Oxygen near the Seafloor Indicator Assessment ).
Changes in upwelling intensity
Within the OSPAR Maritime Area, wind-driven upwelling is particularly important along the western margin of Iberia in Region IV, where it exerts strong control over productivity, with wide-ranging effects across the trophic levels of the region’s marine ecosystem. The changes in productivity have been described for the last 1 000 years (e.g. Abrantes et al., 2011), but there is contradictory evidence as to the direction of changes in recent times and the projections for the future. Recent intensification in upwelling has been described for the African coast (McGregor et al., 2007), but other analyses of wind data have shown differences in trends at sub-regional scale (Alvarez et al., 2008; Pérez et al., 2010; Santos et al., 2011), a general weakening in the region (Pardo et al., 2011; Siemer et al., 2021) or no clear evidence of upwelling decrease (Barton et al., 2013; Benazzouz et al., 2015). Indeed, estimations based on regional climate models predict an increase in upwelling-favourable winds in the region (Casabella et al., 2014). Satellite-derived chlorophyll series have not revealed a consistent trend through the region (Bode et al., 2011; Alvarez et al., 2012: Benazzouz et al., 2015), but in situ determinations near the coast suggest large year-on-year variations (Bode et al., 2011), or even increases at decadal time scales (Pérez et al., 2010).
Marine heatwaves
Marine heatwaves are defined as periods of extreme high sea surface temperature relative to the long-term mean seasonal cycle (Hobday et al., 2016). Such events can have negative impacts on marine ecosystems, including mortality events and plankton blooms and, over longer time scales, can cause shifts in species distribution and declines in commercial fishery catches (Collins et al., 2019). Marine heatwaves can also extend widely in both geographic (millions of km2) and temporal terms (weeks to months). Collins et al. (2019) have highlighted that large-scale climate models can either amplify or supress the occurrence of marine heatwaves. They report that marine heatwaves doubled in frequency between 1982 and 2016, increasing in intensity by 0,04° C per decade and in spatial extent by 19% per decade. Climate projections suggest that marine heat-waves will become more frequent in the future under most shared socio-economic pathway scenarios and may last significantly longer. The Arctic region is likely to be one of the regions impacted by increased frequency and intensity in future marine heatwaves. There are very limited studies available to date on marine heatwaves in the OSPAR Maritime Area. A recent study in Region II (Wakelin et al., 2021) found evidence of widespread heatwaves in the southern North Sea over the last 30 years, but no evidence of a trend in extent or strength. Even during this period of general warming, they also found no significant trend in winter cold snaps.
Activities | State |